1. Obesity: Epidemiology, Etiopathophysiology, and Early Development
Obesity, defined as a body mass index (BMI) ≥30.0 kg/m
2, is a multifactorial, chronic, and relapsing disease that has spread to pandemic proportions during the last decades
[1][2]. Obesity prevalence has in fact nearly tripled since 1975 and its incidence is expected to increase further in the near future
[1][2][3]. This estimate is not surprising as the incidence of obesity among children is rising steeply
[4] and the condition is usually maintained throughout life
[5]. Notably, in 2016, more than 1.9 billion people (39% of adults) worldwide were overweight (25.0 ≤ BMI < 30.0 kg/m
2) and more than 650 million suffered from obesity (13%). Furthermore, in 2020, 39 million children under the age of 5 were overweight or suffered from the disease
[6]. Obesity is associated with a higher risk of developing over 200 medical complications, including insulin resistance, type 2 diabetes mellitus, hypertension, metabolic syndrome, cardiovascular disease, and several types of cancer. For the above reasons, obesity is recognized as the fifth leading cause of death worldwide and as a major burden for the global healthcare systems
[6][7][8].
From a biological standpoint, obesity results from the inability to ensure energy homeostasis, an impairment referred to as energy balance dysfunction. This concept is often simplistically ascribed to excessive energy intake (eating) and low energy expenditure (physical activity), hence to an “unhealthy lifestyle”. Nonetheless, the etiology of obesity is complex and multifactorial
[9]. According to the Foresight Study, multiple environmental (i.e., food industry, pollution, education, culture, access to healthcare), psychological (individual and social), and biological (genetic, epigenetic, endocrinological) factors not only contribute to determine the obesity risk, but also positively and negatively influence each other in triggering the disease and its morbidity
[9]. Eventually, the interplay between these causal factors results in a dysfunctional regulation of the energy balance, hence abnormal energy intake and expenditure.
The pathophysiology of obesity involves multiple organs. The chronic positive energy balance results in an excessive accumulation of lipids within the adipose depots and in different cytotypes. This phenomenon is responsible for adipose tissue expansion, inflammation, and lipotoxicity and deeply compromises several organ functions
[7][10][11][12][13]. For example, obesity-related lipotoxicity and chronic inflammation may result in non-alcoholic fatty liver steatohepatitis; skeletal muscle dysfunction, i.e., sarcopenic obesity
[12][14]; and pancreatic β-cell impairment
[15]. Overnutrition and obesity are associated with inflammation and endoplasmic reticulum stress in the mediobasal hypothalamus, which hosts the centers regulating the energy balance
[16]. Organ dysfunction in turn impairs whole-body energy homeostatic abilities and triggers a vicious cycle that underpins the chronic and relapsing nature of obesity
[1][7].
The energy balance is controlled by the central nervous system (CNS)
[17][18][19][20]. The master regulator is the hypothalamus, where all signals from other brain areas and from the periphery are integrated and translated into specific behavioral, autonomic, and endocrine outputs
[17][18][19][21][22]. The crucial role of the CNS in obesity susceptibility is documented by recent genome-wide association studies that implicated pathways related to synaptic function, extracellular matrix composition, glutamate signaling
[23], and brain G protein-coupled receptors as key factors governing BMI variations
[24].
The maturation of the central neural circuitries involved in energy balance control is not completed at birth but also occurs during early postnatal life. In mammals, postnatal ages are denoted by critical developmental periods during which organs and neural systems are highly plastic. In this timeframe, adverse nutritional, social, and environmental cues may program body metabolism to maximize energy accrual to cope with hostile conditions. Accordingly, the prevalence of obesity is higher among individuals exposed to early life stress (ELS) during both the pre- and postnatal periods
[25].
In rodents, thermogenic brown adipose tissue (BAT) is present at birth to sustain pups’ survival, whereas white adipose tissue (WAT) progressively develops during the first postnatal weeks. This timing may reflect an evolutionary strategy aimed at adjusting metabolic functions to environmental cues, such as maternal care and food availability. Obesity disease is hence rarely due to environmental and/or genetic factors alone and results from the interaction between the individual’s biological characteristics and the environment in which he lives in
[1]. For this reason, obesity is recognized as a preventable disease
[1].
The study of the cellular and molecular mechanisms at the basis of the postnatal differentiation of the energy homeostatic circuitry may shed light on the etiopathogenetic basis of several forms of obesity and may offer new targets for intervention and prevention. Given the strong association between disorganized/inconstant maternal care and obesity risk
[26][27], the identification of the biological pathways and mediators of such a link is of relevance. The oxytocin system is a highly promising candidate, given its role in maternal bonding, response to stress, and feeding behavior
[28][29][30]. Researchers report evidence from the literature documenting the effect of ELS (specifically postnatal stress induced by disorganized/inconstant maternal care) on obesity vulnerability, with a particular focus on oxytocin (Oxt) and leptin (Lep) roles in rodent models. Researchers emphasize the existing gaps in the literature and highlight promising research directions worthy of exploration.
2. Early Life Stress
Clinical observations and studies by authoritative psychiatrists and pediatricians, such as John Bowlby and Donald Winnicott, emphasized the crucial role of early life experiences, in particular the relationship between the infant and the mother (or caregiver), in shaping individuals’ psychological health
[31][32]. Specifically, a disorganized care attitude toward the offspring results in abnormal attachment/bonding to the parental figure and in the development of dysfunctional behaviors (e.g., depression, drug addiction, and eating disorders) in adulthood
[26][27][30][31]. During early life, parental–offspring interactions exert a critical influence on offspring’s developmental trajectories
[33]. In animal models, ELS is usually reproduced by maternal separation (MS), which consists in separating pups from their dam for 180 min per day, or by limiting the nesting and bedding material (LN), a procedure that results in disorganized maternal care and hence stress among pups
[34][35]. Maternal care anomalies lead to abnormal developmental courses underlined by very complex and poorly characterized neuroendocrine cascades
[36]. Growth in an unpredictable environment, where maternal care is not consistent, may result in a neurometabolic programming aimed at maximizing energy accrual and minimizing its waste, a possible adapting strategy to face hostile conditions. As a result, the rewarding value of feeding may also be abnormally enhanced
[21].
In adult humans and experimental animals, acute stress suppresses appetite while chronic stress often leads to weight gain due to the persistent overactivation of the hypothalamic-pituitary-adrenal (HPA) axis and to opioid release; both systems promote “comfort eating” as a coping strategy
[18]. Although the effects of acute and chronic stress on eating behavior and metabolic health have been extensively studied in adults, the mechanisms through which ELS eventually affects them in adulthood are unclear
[26] (
Table 1). Exposure to stress during early life is associated with overweight and obesity in adult humans
[26][27]. ELS is known to negatively impact neurobiological, cognitive, social-emotional, behavioral, and physical development in humans and animal models. In the timeframe characterizing early development, known as the critical period, the infants’ brain is extremely plastic. Intense phenomena of pruning and synaptogenesis are necessary to shape neural circuit and to complete brain maturation, which concludes only after adolescence
[33]. In this developmental process, a crucial role is also played by glial cells, which actively modulate synaptic formation, pruning, neurovascular coupling, and phagocytosis
[37]. Glial cells also act as metabolic sensors, integrating a multitude of signals to adapt to the environment
[38]. This period of high plasticity is deeply influenced by life experiences and is characterized by a marked vulnerability to negative stimuli, which can shape the neural network
[33][36]. However, relatively little is known about how these critical periods impact the development of peripheral organs (e.g., adipose tissue) and their crosstalk with the CNS. The study of obesity etiopathogenesis requires not only a longitudinal approach that explores in depth early life events, but also a comprehensive research strategy investigating the crosstalk between the CNS and peripheral organs.
In the study of early adversity determined by abnormal infant care, particularly pertinent is the research on the neurohormone Oxt. Produced by neurons located in the hypothalamic paraventricular (PVN) and supraoptic (SON) nuclei (Figure 1A-E), Oxt plays a pivotal role in the regulation of a variety of behaviors including social, emotional, sexual, eating, and addiction behaviors [21]. Oxt is produced by both magnocellular and parvocellular neurons: the formers are contained in PVN and SON and mainly project to the neurohypophysis where Oxt is released into the circulation, while the latters, mainly contained in the PVN but also scattered in other hypothalamic and extrahypothalamic areas, project to different hindbrain regions e.g., solitary tract nucleus [39][40]. Interestingly, PVN and SON magnocellular Oxt neurons develop axon collaterals projecting to forebrain limbic regions (e.g., prefrontal cortex, nucleus accumbens, anterior and central amygdala, bed nucleus of the stria terminalis (BNST), hippocampus) (Figure 2A–F). This finding has only been described in advanced vertebrates and is believed to have developed together with the social and emotional behavioral complexity of species.
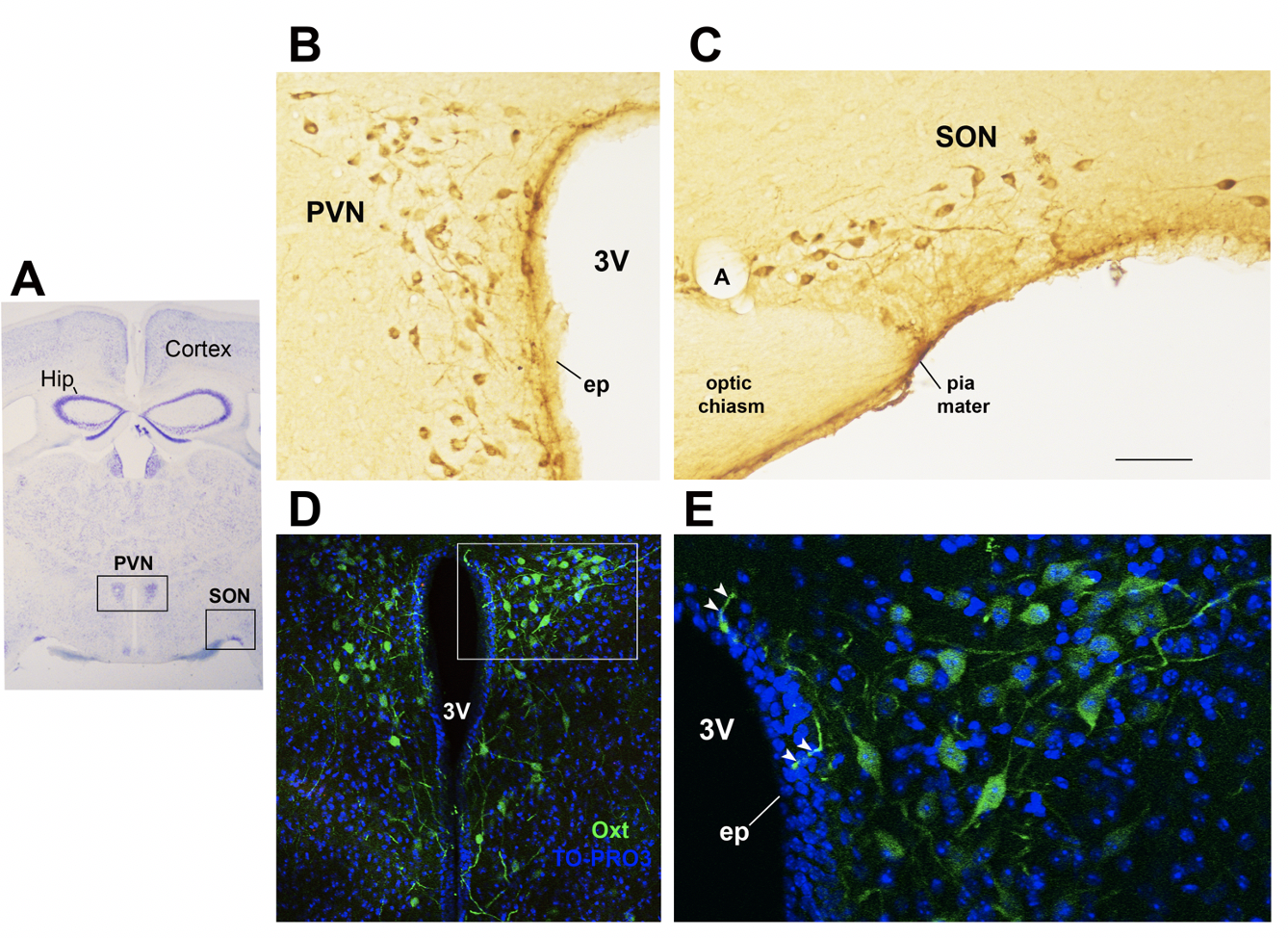
Figure 1. Oxytocinergic neurons in the paraventricular (PVN) and supraoptic (SON) hypothalamic nuclei. (A) Light microscopy (LM): Nissl-stained brain coronal section of the region corresponding to bregma −0.94 mm; PVN: hypothalamic paraventricular nucleus; SON: hypothalamic supraoptic nucleus; Hip: hippocampus. (B) LM: peroxidase immunohistochemistry of oxytocin (Oxt)-positive neurons in proximity of the ependymal layer (ep) of the third ventricle (3 V) in the PVN. (C) Peroxidase immunohistochemistry of Oxt neurons in the SON; A: artery. (D) Double-label confocal microscopy of Oxt neurons (green) and cell nuclei (blue TO-PRO3 staining) in the PVN. Panel (E) is an enlargement of the area framed in (D), showing Oxt-positive neurons and their projections (arrowheads) reaching and contacting the ependymal cells and the cerebrospinal fluid of the third ventricle (3 V). All figures refer to a 6-month-old male C57BL/6 mouse. Bregma reference sections from “The Mouse Brain Atlas”, Paxinos and Franklin (2001). The scale bar is included in C only and corresponds to different μm in each figure as follows: in (A): 1500 μm; in (B,C): 45 μm; in (D): 120 μm; in (E): 40 μm. All figures are original.
Oxt stimulates maternal care, maternal–infant attachment, and social bonding and can attenuate the response to stress, anxiety, and depression [21][28]. It also has a crucial role in the regulation of the stress system by reducing HPA activity and by supporting the parasympathetic nervous system [28][41][42]. Recent works on the neurobiological basis of attachment, coupled with studies on children adopted from orphanages, suggest that there may be a sensitive period for the development of Oxt–dopamine connections (particularly in the nucleus accumbes of the striatum), which exerts enduring effects on the neurobiology of social relationships [41], for instance, by strengthening the ability to buffer stress [42].
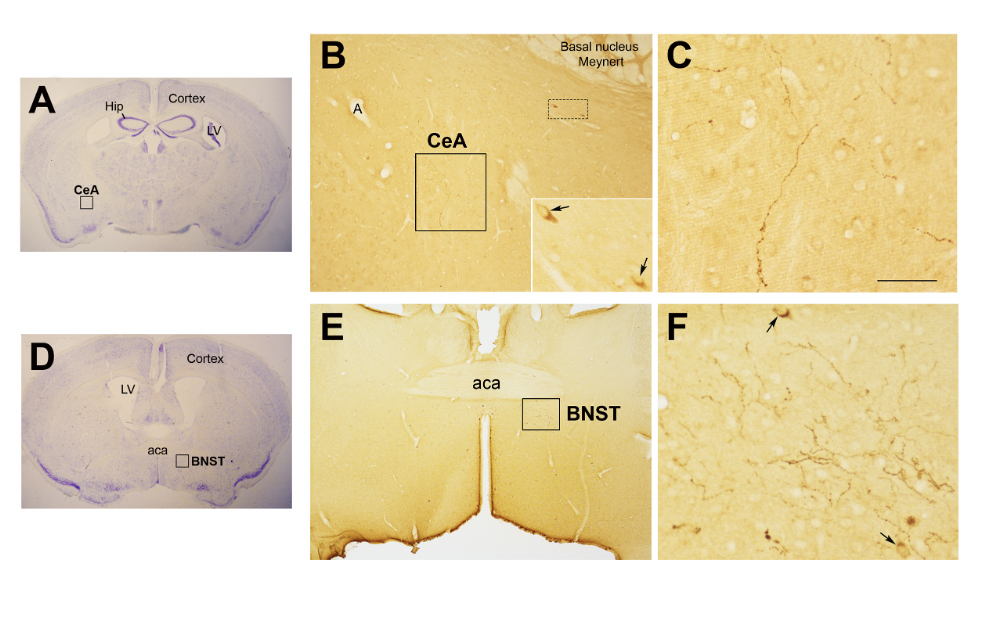
Figure 2. Oxytocinergic projections in the central nuclei of the amygdala (CeA) and in the bed nucleus of the stria terminalis (BNST). (A) Light microscopy (LM): Nissl-stained brain coronal section of bregma −0.94 mm; Hip: hippocampus; LV: lateral ventricle; CeA: central nuclei of the amygdala. (B) LM: peroxidase immunohistochemistry of oxytocin (Oxt), showing oxytocinergic fibers (framed area) and two parvocellular Oxt neurons (dotted framed area) in the CeA. Inset: enlargement of the dotted framed area, where Oxt-positive neurons are indicated by arrows. (C) Enlargement of the area framed in (B), rich in Oxt-positive fibers. (D) LM: Nissl-stained brain coronal section of the bregma 0.02 mm; BNST: bed nucleus of the stria terminalis; aca, anterior commissure. (E) LM: peroxidase immunohistochemistry of oxytocinergic projections in the BNST. (F) Enlargement of the area framed in (E) showing Oxt neurons (arrows) and Oxt projections in the BNST. All figures relate to a 6-month-old male C57BL/6 mouse. Bregma reference sections from “The Mouse Brain Atlas”, Paxinos and Franklin (2001). The scale bar is only specified in C and corresponds to different μm in each figure, as follows: in (A,D): 2300 μm; (B): 200 μm, inset 50 μm; in (C): 50 μm; in (E): 300 μm; in (F): 50 μm. All figures are original.
Interestingly, Oxt also attenuates addictive behaviors and inhibits appetite [41]. Consistent with these data, there is a mounting body of evidence pointing at Oxt role in promoting weight loss and ameliorating obesity-related metabolic dysfunctions [29][43][44][45]. Intranasal Oxt administration is currently being tested for the treatment of obesity as this route facilitates an increase in the central concentrations of the nonapeptide through channels surrounding the trigeminal and olfactory nerve fibers [46][47][48]. Considering the complex and multiple functions of Oxt, which affects aspects as diverse as mother–infant bonding, eating behavior, and stress response, its potential role in determining the impact of ELS on eating behavior and metabolic health deserves further investigation.
5. Oxytocin System: Early Development and Impact of Early Life Stress
Rodent studies have demonstrated that early adverse experiences may have a strong impact on the way the Oxt system is shaped. Oxt neurons progressively increase from PND 2 to 21, reaching maturation by the second postnatal week
[49]. In contrast, Oxt axons reach their targets only in early adulthood, when Oxtr is already widely expressed in various regions. Specifically, Oxtr is detected in females from embryonic day 14 and in males from PND 2
[40]. Importantly, Oxt-synthesizing neurons play a fundamental role in their own maturation: local Oxt release activates Oxtr expressed on Oxt neurons, resulting in further Oxt discharge. These data explain how deeply early variations in Oxt levels can impact the maturation of Oxt neurons
[21][49][50]. It is hence possible that postnatal environmental stimuli affect Oxt production and the development of the Oxt system, resulting in the exclusive retention of functional pathways necessary to face external conditions. This would also explain the interindividual variability of Oxt neuron projections revealed by Oxt system mapping
[51]. Notably, the different spatio-temporal Oxtr expression patterns described in males and females during development suggest a distinct and sex-specific sensitivity to Oxt levels during this critical period
[40].
Altered Oxtr expression and binding in response to changes in maternal care were described in several brain regions in different animal models [36][52]. Maternal high licking and grooming (LG) result in increased Oxt expression at PND13 while low LG leads to reduced Oxtr protein levels and receptor binding in several females’ brain regions (e.g., PVN, central nucleus of the amygdala) [36][52][53]. Furthermore, while one study detected a higher number of Oxt-positive cells in the PVN of adult male mice exposed to MS [54], other investigations with a similar protocol reported a lower number of these cells in the SON and PVN [42][55][56]. In addition, higher Oxtr expression and a higher number of Oxt projections to the basolateral amygdala were described in male mice exposed to MS [56]. Early adversity usually results in reduced circulating Oxt [42]. However, Oxt levels may actually rise in response to prolonged exposure to adverse stimuli, possibly to protect the system from the harmful effects of stress [42].
A meta-analyses performed by Ellis and colleagues documented lower plasma OXT levels and reduced or negative response to OXT intranasal administration among individuals who experienced childhood adversity [57]. In addition, an unsecure attachment style was associated with lower OXTR expression in the peripheral blood mononuclear cells of women . Based on this evidence, early experience shapes the adult Oxt system and manipulation of maternal care from infancy confers enduring changes in the Oxtr expression profile, a phenomenon whose mechanisms and implications are not fully elucidated. In addition, early stress in childhood may be produced by overexposure to blue light of digital device screens affecting sleep patterns in children as young as 2 years old.
Recent studies documented the association between the alteration in the Oxt system induced by ELS and the development of cardiovascular diseases
[58][59][60]. However, little is known about the link between Oxt, ELS, and metabolic health. While data on the relationship between ELS and Oxt and between ELS and metabolic health are scanty, evidence linking ELS to metabolic health, focusing on the Oxt system, is not documented in the literature. Specifically, it is unclear how ELS induces changes in the action of and sensitivity to Oxt and how these changes influence adipose tissue development, energy balance, metabolic health, and feeding behaviors in adulthood (
Table 1). The sex-dependent differences in the Oxt system may affect the adipose proliferative niches (hence, adipose tissue development) and may partly contribute to the distinctive fat mass distribution and susceptibility to obesity comorbidities described in males and females. Furthermore, while the effect of Oxt manipulation in early development has been widely investigated
[49], little is known about the function of endogenous Oxt at such a time
[30]. Several lines of research have demonstrated how spatio-temporal Oxtr expression varies in response to environmental cues, especially during critical periods. It is widely accepted that Oxtr may be a developmental plasticity gene that acts as a transducer of the social environment to finetune the experience-dependent plasticity of the social brain
[40]. Therefore, Oxtr expression and distribution, as opposed to circulating Oxt levels per se, may be of particular significance. As noted above, Oxt influences reward-related behaviors in different ways: while it reduces motivated behavior toward palatable food, it modulates social rewards and attention-orienting responses to external social cues
[61]. It is reasonable to hypothesize that dysfunctional social attachment to the parental figure during early development (MS or LN) alters the oxytocinergic system maturation such that the reward response to feeding prevails on the reward response to social cues. This paradigmatic model may be employed not only to study obesity vulnerability, but also psychiatric diseases, such as eating disorders, depression, and addiction. Indeed, the limitations related to the use of animal models for the study of such complex diseases should be acknowledged. Growth in an unpredictable environment, where maternal care (feeding) is not constantly ensured, may result in a neurometabolic programming that maximizes energy accrual and minimizes its waste. As a result, besides the enhanced reward value of food, the ability to store energy to cope with an adverse environment may also be increased (
Figure 3). Deeper investigation of the biological underpinnings of ELS-induced metabolic and eating behavior abnormalities is warranted to characterize unexplored mechanisms responsible for obesity and to identify novel preventive and/or therapeutic strategies.
Table 1. Outstanding questions to be addressed.
Figure 3. Schematic representations of the pathways linking early life stress to vulnerability to adult obesity. (A) Impact of environmental stimuli on the early postnatal development of various organs (e.g., brain, liver, brown and white adipose tissues, and skeletal muscle). (B) Oxytocin (Oxt), produced in the paraventricular nucleus (PVN) and supraoptic nucleus (SON) of the hypothalamus, regulates maternal bonding, stress response, and energy homeostasis. Oxt receptor (Oxtr) is highly expressed in white adipose tissue. Adipocytes are the main source of leptin (Lep), the master energy balance regulator, whose receptor (Lepr) is expressed in Oxt neurons. (C) Early adverse experiences may affect brain development, metabolically relevant organs differentiation, and the Oxt and Lep systems maturation. In this condition, dysfunctional neurometabolic programming and homeostatic and hedonic feeding centers maturation may occur, ultimately enhancing the vulnerability to obesity in adulthood. VMH: ventromedial, DMH: dorsomedial, LH: lateral hypothalamic nuclei; ARC: arcuate nucleus; NTS: nucleus of the solitary tract; CC: cerebral cortex; Nacc: nucleus accumbens; BNST: bed nucleus of the stria terminalis; Amyg: amygdala; VTA: ventral tegmental area.