Potassium ion channels are a class of transmembrane proteins involved in maintaining the electrical microenvironment of cells. In cancers, these proteins are known to play a significant role in the development of cancer hallmarks like proliferation, invasion, and adaptive drug resistance. Malignant central nervous system (CNS) cancers are notoriously difficult to treat, with just one-third of patients surviving five years post-diagnosis. Their location within the brain and brainstem presents several challenges to successful treatment, including surgical inaccessibility and designing effective therapies that pass the brain’s protective barrier. Furthermore, high-grade CNS cancer is also prone to recurrence, and the secondary tumours that arise are highly resistant to treatment.
1. Introduction
Intercellular communication is fundamental to maintaining homeostasis in a biological system, and this exchange is facilitated by proteins known as ion channels. Ion channels comprise one or more subunits that arrange to form a specialised pore, allowing ion flux across cellular membranes. Over 300 unique ion channel subtypes exist in human cells
[1], each classified based on their selectivity for specific metal ions (K
+, Na
+, Ca
2+, Cl
−, etc.) and gating mechanisms. Potassium ion channels describe a family that comprises more than 90 proteins and include calcium- and sodium-activated potassium channels (K
Ca, K
Na), inwardly rectifying potassium channels (K
ir), two-pore domain potassium channels (K
2P), and voltage-gated potassium channels (K
v or VGKCs)
[2]. The selective flow of metal ions across cell membranes enables intercellular signalling by regulating the membrane voltage potential
[3]. Cellular voltage can become more positive (depolarisation) or negative (hyperpolarisation) in response to environmental stimuli.
One way that ion channels contribute to maintaining homeostasis is through regulating the resting membrane potential (RMP) of cells. The RMP of a cell refers to the difference in electrical potential across the plasma membrane when the cell is in a non-excited state (described in
[4]). This is maintained primarily by the Na
+/K
+ ATPase pump, which pumps three Na
+ ions out of the cell and two K
+ ions into the cell to generate a concentration gradient across the plasma membrane. The RMP varies widely based on cell type and localisation; for example, mature astrocytes rest at approximately −80 mV
[5], myocardial cells at −90 mV
[6], and non-excitable red blood cells at −10 mV
[7], while cancer cells, such as glioblastoma (GBM), typically exhibit much more depolarised RMP’s (−20 to −40 mV)
[8]. A dynamic membrane potential allows excitable cell types (neurons and muscle cells) to experience rapid and significant changes in polarity, generating action potentials. However, the importance of ion channel proteins extends beyond action potential propagation, as they also play a significant role in non-excitable cell physiology, particularly regulating the cell cycle. The cell cycle describes a process where after cell division, the cell enters a growth phase (‘G
1’), then undergoes DNA duplication (‘S’), followed by a second growth phase where the DNA is checked for errors (‘G
2’) before mitosis (M). Mediated by ion channels, cyclic changes in voltage potential of the cell membrane accompany physical cellular changes during each phase.
Aberrant ion channel expression and dysfunction can give rise to various disease states, known as channelopathies
[9]. Further, the close association between ion channels and cancer has led to the development of a new classification: oncochannelopathies
[10]. Although not all cancer hallmarks are inherently linked to ion channel mutations, dysfunction, or abnormal expression, these can certainly contribute to disease progression. These hallmarks include unlimited proliferation, increased invasion and migration, and evasion of apoptosis
[11]. Further, potassium ion channels (primarily K
Ca) promote recruitment of existing vasculature (angiogenesis) and the formation of entirely new vasculature (vasculogenesis)
[12][13][14]. This is essential in providing oxygenated blood carrying essential nutrients to both healthy and malignant cells.
2. Potassium Ion Channels
The potassium ion channel family is the largest of all ion channel classes and comprises four subfamilies: voltage-gated (VGKC), sodium and calcium-activated (K
Na, K
Ca), inward-rectifying (K
ir), and two-pore domain (K
2P) potassium channels
[15]. The channels in each subfamily differ based on their domain structure, gating mechanisms and function.
Figure 1 provides a schematic representation of the general structure of these protein subfamilies. VGKC, K
Na and K
Ca channels share a basic 4 α-subunit structure with a single pore-forming region (TM5–TM6), while K
ir channels consist of only two α-subunits. K
2P channels possess two separate pore-forming domains and a four α-subunit structure. Furthermore, these multimeric proteins are often associated with one or more auxiliary β-subunits (encoded by genes
KCNE1–
5) that further influence channel gating
[16].
Figure 1. General schematic structure of the four major potassium channel subfamilies. TM1–TM6 represent transmembrane segments and ‘P’ represents pore-forming regions between subunits. VGKC and K
Ca channels share a common four subunit structure (TM1–TM4) that comprises the selective voltage-sensing domain
[17]. TM5 and TM6 correspond to the pore domain found in all K
+ channels
[18]. K
Na channels also follow this basic transmembrane structure but encode additional residues within the C-terminal region that regulate K
+ conductance
[19]. K
ir channels have two transmembrane domains and a single pore-forming region between them. K
2P channels are comprised of four TM domains and two separate pores. Modified from
[20].+++: Positively-charged side; ---: Negatively-charged side.
VGKCs conduct K
+ ions at a fast rate due to their highly conserved pore domain, which is closely linked to their function
[17]. The pore consists of a channel gate and a selectivity filter, preventing other metal ions from entering the channel. K
+ ions move in a single file arrangement through the channel pore, where the cumulative repulsion of charge-charge interactions between the positively charged K
+ ions largely drives their movement
[21]. VGKCs remain closed until they sense a depolarisation at the cell membrane, at which point the protein undergoes structural changes, resulting in an open conformation
[22][23]. This allows K
+ ions to flow through the pore and into the cell, until the membrane is repolarised, and the channel becomes inactivated. Because of their functional properties, VGKCs are essential to numerous biological processes that depend on sensing changes to membrane potential. This includes potential propagation
[24], cellular growth and changes to volume
[25], apoptosis regulation
[26], and cardiac muscle function
[27].
While K
Ca and K
Na channels may share structural parallels with their voltage-gated counterparts, they are functionally different. K
Ca channels are not voltage-dependant, and instead are gated by intracellular Ca
2+. K
Ca channels are divided into three subfamilies: small conductance (SK), intermediate conductance (IK) and big conductance (BK) channels
[28]. SK and IK channels are activated when calmodulin binds to their receptor domain in response to low intracellular concentrations of Ca
2+ (~0.5 μM). BK channels differ in that they contain an additional transmembrane domain, allowing for the presence of two high-affinity Ca
2+ binding sites
[29][30]. Thus, BK channels are directly activated and opened by Ca
2+ binding, bypassing the need for calmodulin.
K
ir channels are primarily responsible for K
+ influx and have a range of physiological roles depending on their tissue distribution. These channels remain open at rest and therefore play a key role in maintaining the RMP by regulating membrane voltage to stay close to the K
+ Nernst potential (−60 mV to −90 mV). Additionally, K
ir2.1 has been shown to play important roles in muscle contraction and early bone development
[31][32].
Finally, K
2P channels comprise an essential group of proteins in supporting neuronal function. These channels are unique in that they comprise two distinct pore domains within a singular protein. They are often referred to as ‘leak’ channels, as they produce relatively small, non-inactivating K
+ currents across a wide range of membrane potentials
[33]. K
2P channel currents can be influenced by a number of stimuli, including kinases, lipids, G-proteins, changes in pH, mechanical force, and interactions with other proteins
[34].
3. Malignant CNS Cancer
In 2020, the worldwide incidence rate for primary malignant CNS tumour diagnosis was 3.5 per 100,000
[35]. Gliomas make up many of these cases, accounting for approximately 78.3% of malignant CNS cancer diagnoses in the United States
[36]. With hundreds of thousands of new cases diagnosed each year, there is a significant burden placed on the healthcare system, and individual patients, to treat this disease. For example, the median expenditure for an individual undergoing treatment for HGG is $184,159.83, with radiation therapy accounting for most of this cost
[37]. Only one-third of malignant CNS cancer patients will reach the 5-year survival point post-diagnosis
[36]. This high mortality rate is largely due to inadequate treatment protocols.
GBM, diffuse midline glioma (DMG), and anaplastic astrocytoma are classified as HGG. GBM carries the worst prognosis of all adult primary CNS cancers, with a 5-year survival rate of less than 5% and an average survival time of just 15 months
[38]. GBM accounts for almost 50% of all malignant primary CNS tumours
[36] and the average age of GBM patients at diagnosis is 64 years. The cell type of origin for GBM has remained a debate for decades, with a current consensus that neural stem cells (NSCs), NSC-derived astrocytes and oligodendrocyte precursor cells (OPCs) are all cells of origin
[39]. Current treatment strategies have remained unchanged for many years and are considered primarily palliative, beginning with surgical resection of as much of the tumour mass as is feasible (
Figure 2). Due to GBM’s highly infiltrative and diffuse growth pattern, it invades surrounding healthy brain parenchyma to a high degree, making whole tumour debulking impossible
[40]. Surgery is then followed by combined adjuvant radiotherapy and temozolomide treatment, which has shown to increase the 2-year survival rate from 10.4% to 26.5%
[38]. Even with these combined efforts, the prognosis for patients with GBM remains extremely poor, with treatment often adding mere months to their survival time.
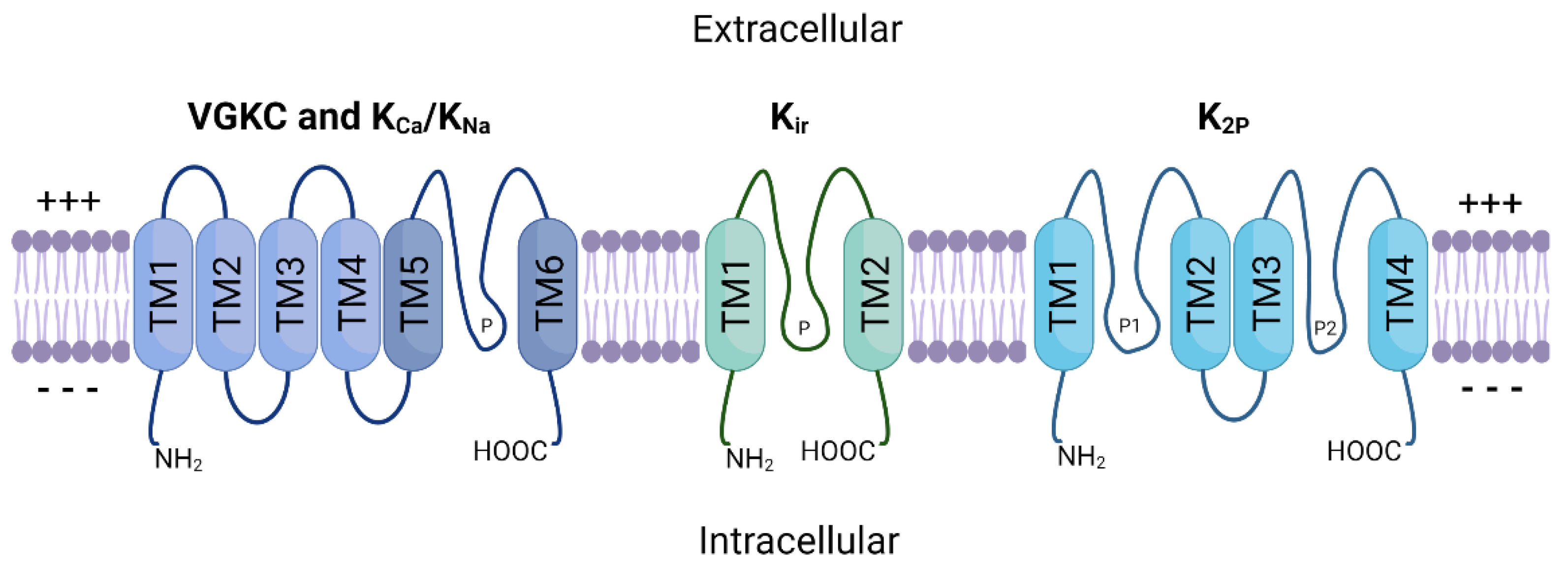
Figure 2. Overview of the current ‘gold standard’ GBM treatment strategy.
Panel 1: Debulking surgery is performed to remove as much tumour mass as possible. Due to extensive infiltration, some metastatic cells inevitably remain.
Panel 2: Combined radiotherapy and temozolomide (TMZ) treatment is administered over several months.
Panel 3: Remaining malignant cells (primarily GSCs) overcome and adapt to TMZ treatment, continuing to proliferate.
Panel 4: Inevitably, tumour recurrence and metastasis occur, resulting in eventual death.~: Median survival.
3.1. Targeted Therapies
As discussed, current treatment methods for HGG are non-curative, hence the pressing need to develop novel and improved treatment strategies. Several challenges exist in treating HGG through traditional methods
[41]. Firstly, their location within the brain or brainstem reduces accessibility for complete tumour resection and increases the likelihood of side effects from tumour growth into healthy brain tissue. In addition, HGG tumours are highly heterogenous, and often constitute multiple different sub-populations of cells within a single tumour. Furthermore, many existing chemotherapeutics do not cross the blood-brain barrier (BBB) and present many undesirable side effects to patients due to their non-targeted mechanisms of action. Targeted therapy represents an attractive solution to these problems, through which malignant cells are specifically targeted, bypassing healthy brain tissue and reducing off-target effects. However, many targeted therapies have tried and failed to improve survival in patients with HGG, despite promising results in vitro and in vivo
[42].
Epidermal growth factor receptor (EGFR) and its variant EGFRvIII are commonly amplified in GBM, with over half of primary GBM cases showing upregulated EGFR
[43]. This has led to a number of clinical trials directed at EGFR inhibition to prevent or slow GBM tumour growth. Rindopepimut was developed as a novel ‘tumour vaccine’ to target the EGFRvIII mutation site and was put through phase I and II clinical trials
[44]. Primary GBM patients whose tumours expressed EGFRvIII were recruited and treated with a combination of rindopepimut and temozolomide as standard. Patient outcomes varied across the group but were generally more favourable than matched historical controls. Trial participants overall survival ranged from 22–26 months compared to 15 months with TMZ treatment alone. This represented a promising breakthrough in targeted therapy for HGG. Unfortunately, the rindopepimut and temozolomide combination therapy failed to improve survival in a phase III trial
[45].
Factors influencing angiogenesis are also primary targets for HGG treatment, as reducing blood flow to malignant cells can arrest growth and invasion. Yet, the anti-angiogenic agent cediranib, a VEGF receptor tyrosine kinase inhibitor, was ineffective in influencing GBM patient outcomes in phase III trials
[46]. While bevacizumab, a monoclonal antibody that binds to VEGF-a, also failed to improve overall survival in several phase III trials
[47][48]. There are several potential reasons underpinning the failure of targeted therapies in HGG treatment. These include inadequate drug penetration to the tumour site, tumour heterogeneity, lack of tumour dependence on target proteins, clonal evolution, and antigen escape
[42]. Further, because HGG cells possess a high degree of plasticity, reversibly shifting between multiple unique ‘states’
[49] HGG cells bypass targeted therapies by adapting alternative growth pathways and maintaining proliferation. As ion channels are primary drivers of cellular plasticity
[50][51], inhibiting these proteins could reduce resistance to targeted therapies.
3.2. Cellular Plasticity and Drug Resistance
Both healthy and malignant neural cells are highly plastic, a significant barrier preventing the success of targeted HGG therapy. Sturm et al.
[52] identified the six distinct states, differentiated by their DNA methylation patterns, between which HGG cells continually and reversibly transition. This plasticity underpins adaptive drug resistance, in which alternative signalling pathways are activated in response to the targeted blockade of integral proteins
[53][54][55]. This process largely relies on glioma stem cells (GSCs), unique malignant cell populations capable of forming heterogenous HGG tumours. Many cancerous cells that remain following standard HGG treatment can be classified as GSCs. They are characterised by their heightened resistance to drugs, unlimited proliferation, and multipotent differentiation
[56].
GSCs are believed to be responsible for HGG’s adaptive response to treatment by developing alternative oncogenic pathways that bypass the targeted proteins or pathways
[57][58]. For example, TMZ resistance is common in GBM tumours, and GSCs are believed to be primarily responsible for this
[59]. Firstly, whole genome sequencing of matched primary and recurrent GBM tumours showed common deleterious mutations in
TP53 that originated from GSC populations
[60][61]. Mutations in
TP53 are common in recurrent GBM, and linked to heightened invasion, migration, proliferation and GSC propagation
[62].
It has been established that ion channels, including potassium ion channels, are key proteins in stem cells, including GSCs. Following treatment with the K
Ca3.1 antagonist TRAM-34, GSC cell mobility and migration was significantly reduced
[63].
Similarly, to glioma, MB tumours are largely heterogenous and diverse, exhibiting various cell types, including stem cell-like populations (MBSCs)
[64]. Single-cell analysis of MB tumours following vismodegib treatment revealed an increase in the population of MBSCs, which express the oncogene,
SOX2 [65].
SOX2 plays a significant role in cancer cell stemness and is often overexpressed in the stem-cell populations of both HGG and MB
[66].
4. Potassium Ion Channels in Malignant CNS Cancer
4.1. High-Grade Glioma
The K
+-regulating ion channel family is perhaps the most well understood regarding HGG pathophysiology and tumourigenesis, although several published studies have conflicting results. For example, BK channel overexpression has been identified in biopsy samples of GBM patient tumours, with the level of overexpression directly related to increasing malignancy grade
[67]. The importance of BK channels was further solidified by the discovery of a novel splice isoform of the protein (gBK channels) that is exclusively expressed in GBM. The gBK channels appear to be directly involved in the cellular volume/size changes required for invasion of healthy brain parenchyma. Increased cytosolic Ca
2+ concentration, for example via treatment with menthol, activates gBK channels and facilitates greater GBM cell migration and invasion
[68]. While siRNA-induced knockdown of gBK channels in GBM does not change proliferation rates
[69], various BK channel inhibitors can decrease cellular proliferation and increase tumour shrinkage in GBM cell cultures
[70]. Conversely, BK channel activators were shown to reduce glioma cell migration by as much as 50%, suggesting that increased BK channel activity may, in fact, reduce glioma invasiveness
[71].
Inwardly rectifying K+ (K
ir) channels have also been implicated in GBM progression and tumourigenesis. K
ir channels are found in abundance in normal glial cells, where they produce large, inwardly rectifying K
+ currents that stabilise the membrane potential to approximately −90 mV
[72]. However, when glial cells become malignant, the cellular membrane becomes depolarised, increasing up to −20 mV
[8]. Further studies showed that K
ir channels are expressed in GBM but are localised to the nucleus rather than the plasma membrane
[73]. This reduces the effect that K
ir channel function can have on overall cellular membrane potential. It may also contribute to epileptic seizures in many patients with GBM, as mislocalisation of K
ir proteins can result in increased extracellular K+ ion concentration, which is associated with spontaneous seizures
[74]. Thus, although K
ir channels may not be as directly related to tumourigenesis as some other ion channel subtypes, they may represent potential targets in treating GBM-induced seizures.
4.2. Low-Grade Glioma
Compared to HGG, there is significantly less information available regarding how potassium ion channels contribute to LGG disease progression. However, a few studies have explored how specific potassium ion channel subtypes are expressed in LGG. Diffuse grade II
IDH1-mutant gliomas are particularly rare, and often affect young adults. These tumours are particularly heterogenous, displaying multiple different cell subtypes within a single tumour, but the underlying mechanisms of this were not well understood. Augustus et al.
[75] identified that multiple proteins were involved in developing this intratumoural diversity, including K
Ca2.3. In the astrocyte-like tumoural cell populations, electrically active K
Ca2.3 channels were highly expressed, while the oligodendrocyte-like tumour cells showed significantly lower expression. This suggests that K
Ca2.3 may have potential to be used as a marker for astrocyte-like tumoural cells, aiding in patient tumour classification and therapeutic strategies.
4.3. Medulloblastoma
The majority of existing studies targeted at potassium ion channels and medulloblastoma have centred around the K
v11.1 subtype. Huang et al.
[76] were the first to observe that K
v11.1 was overexpressed in MB tumours samples derived from various subgroups compared with healthy brain. K
v11.1 knockdown reduced tumour cell growth in vitro and reduced tumour burden in xenograft mouse models. K
v11.1 was confined inside the cell before the G2 phase of the cell cycle, and upon reaching late G2, it was trafficked to the plasma membrane. Therefore, K
v11.1 knockdown induced G2 arrest, resulting in mitotic catastrophe and cell death. Further studies established that K
v11.1 was specifically enriched in the most invasive subpopulations of MB cells, where it regulated changes to cell shape and volume, thereby facilitating migration
[77]. This process is facilitated by the chloride ion channel, CLIC1, during which both proteins aggregate at lipid rafts to regulate cell volume
[78]. Simultaneous knockdown K
v11.1 and CLIC1 resulted in the suppressed growth/tumour burden in human MB cell lines and fruit fly models. Similarly, loss of the VGKC subtype K
v2.2 results in significantly improved survival in mouse models of MB
[79].
5. Potassium Ion Channels as Therapeutic Targets
Ion channels represent one of the most common targets of currently approved drugs, second only to G-coupled protein receptors
[80]. Several potassium ion channel antagonists, categorised as class III antiarrhythmic agents, have been developed to treat cardiac arrhythmias. These include dofetilide, amiodarone, sotalol, and ibutilide, which act on the K
v11.1, 11.2, and 11.3 VGKCs, respectively, but have known off-target actions other proteins
[81]. It is well established that K
v11 channels are responsible for mediating the heart’s inwardly rectifying K
+ current. Furthermore, missense mutations in these channels can result in type 2 long QT syndrome, triggering arrhythmias
[82]. Due to their integral role in maintaining K
+ homeostasis in the heart, these drugs have potential serious adverse effects and can cause proarrhythmia
[83].
Not all ion channel drugs block channel function. A small group of FDA-approved drugs work by forcing the channel to remain open, allowing increased K
+ ion flow. Most of these drugs, including minoxidil (targets K
ir1.1) and diazoxide (targets K
ir6.2), are classed as vasodilators and are used to regulate blood pressure, while ezogabine targets K
v7.2 to 7.5 and acts as an anti-convulsant
[81]. Alteration of VGKC function via blockade or sustained opening of the channel is of clinical value across several conditions. This is promising regarding CNS cancer treatment because these malignancies appear to be reliant on VGKC function for both growth and metastasis. Furthermore, because many potassium ion channel antagonists are already approved for clinical use in humans, there is potential for rapid clinical translation via drug repurposing. This includes the class III antiarrhythmics, as well as nateglinide and repaglinide, used in the management of diabetes
[84]. When an existing drug is repurposed for a new function, such as cancer treatment, the process involved in receiving FDA approval for the new indication/s is vastly shorter than it would be for an entirely new molecule. With further research, there is potential for developing novel drugs that target specific potassium ion channel subtypes in several CNS cancers.
6. Conclusions
Thanks to the collaborative efforts of numerous research teams, the understanding of the roles of ion channels in tumourigenesis has grown substantially in recent decades. The discovery that ion channels act as crucial drivers of homeostasis via proliferation and cell cycle regulation, and not just as propagators of action potentials, provided the basis from which much of the understanding has grown. It is now understood that ion channels are integral to the processes underlying cellular plasticity, the primary hurdle preventing the successful and curative treatment of HGG. Malignant HGG cells repurpose the endogenously expressed ion channels in healthy glial cells to further their development. Potassium ion channels are perhaps the most well-studied ion channel class in HGG oncogenesis, with many individual studies demonstrating how these cells utilise VGKC, KCa, and Kir channels for enhanced growth and metastasis. Several VGKC-targeting drugs have already received FDA approval, proving that alteration of their normal activity is possible without causing unwanted off-target effects.
Further research should aim to elucidate the roles that potassium ion channels play in CNS cancers and to understand the cellular pathways and downstream proteins they influence. siRNA or CRISPR-mediated knockout studies involving key potassium ion channel subtypes in GBM cell lines would provide further insight into their involvement in key malignant cellular processes. Electrophysiological studies could also help determine whether or not these channels act independently of their current-modifying ion channel functions. Pharmacological inhibition of specific subtypes using repurposed drugs may also demonstrate the potential therapeutic value of these channels in GBM treatment. Finally, the development and use of accurate in vivo models of highly malignant tumours would be of great value, as studies involving cell lines alone can be somewhat limited in scope and potential.