2. MOF-Based Materials for Photocatalytic Nitrogen Fixation
Due to their large pore volumes, adjustable pore sizes, wide light harvesting range, and high densities of exposed catalytic sites, MOFs have been explored for photocatalytic nitrogen fixation. However, it is still in its preliminary stages of development. In addition to pure MOFs, advanced MOF composites and MOF derivatives have also been studied for photocatalytic nitrogen fixation (Table 1).
Table 1. Photocatalytic nitrogen fixation by MOF-based materials.
Photocatalyst |
Light Source |
Sacrificial Agents |
NH3 Yield |
AQY/% |
Ref. |
Pure MOFs |
NH2-MIL-125 (Ti) |
Xe Lamp (300 W, L40) |
None |
12.25 μmolg−1 h−1 |
0.26 (400 nm) |
[69] |
OH-MIL-125 (Ti) |
Xe Lamp (300 W, L40) |
None |
5.04 μmolg−1 h−1 |
/ |
[69] |
CH3-MIL-125 (Ti) |
Xe Lamp (300 W, L40) |
None |
1.39 μmolg−1 h−1 |
/ |
[69] |
UiO-66-UV-Vis |
Xe Lamp (300 W, UV-vis) |
None |
256.60 μmolg−1 h−1 |
/ |
[70] |
UiO-66(SH)2-200 |
Xe Lamp (300 W, L40) |
None |
32.40 μmolg−1 h−1 |
0.45 (420 nm) |
[71] |
MIL-101(Fe) |
Xe Lamp (300 W, full-spectrum) |
None |
50.36 μmolg−1 h−1 |
/ |
[72] |
MIL-100(Fe) |
Xe Lamp (300 W, full-spectrum) |
None |
46.53 μmolg−1 h−1 |
/ |
[72] |
MIL-88(Fe) |
Xe Lamp (300 W, full-spectrum) |
None |
40.04 μmolg−1 h−1 |
/ |
[72] |
MIL-53(FeII/FeIII)-0.1 |
Xe Lamp (300 W, L42) |
K2SO3 |
306.00 μmolg−1 h−1 |
0.12 (420 nm) |
[73] |
Al–PMOF(Fe) |
Xe Lamp (100 mWcm−2, L42) |
CH3OH |
7.06 μmolg−1 h−1 |
/ |
[74] |
MOF-76(Ce) |
Xe Lamp (300 W, full-spectrum) |
None |
34.20 μmolg−1 h−1 |
/ |
[75] |
Gd-IHEP-8 |
Xe Lamp (300 W, AM 1.5 G filter) |
None |
220.00 μmolg−1 h−1 |
2.25 (365 nm) |
[76] |
Gd-IHEP-7 |
Xe Lamp (300 W, AM 1.5 G filter) |
None |
128.00 μmolg−1 h−1 |
1.72 (365 nm) |
[76] |
U(0.5Hf) |
Xe Lamp (300 W, full-spectrum) |
K2SO3 |
351.80 μmolg−1 h−1 |
0.1 (420 nm) |
[77] |
U(0.5Hf)-2SH |
Xe Lamp (300 W, L42) |
K2SO3 |
116.10 μmolg−1 h−1 |
0.55 (420 nm) |
[77] |
NU6(Ce–Hf) |
Xe Lamp (300 W, full-spectrum) |
K2SO3 |
158.4 μmolg−1 h−1 |
0.65 (380 nm) |
[78] |
MOF composites |
ZIF-67@PMO12 |
Xe Lamp (300 W, full-spectrum) |
C2H5OH |
39.40 μmolg−1 h−1 |
/ |
[79] |
ZIF-67@PMO11V |
Xe Lamp (300 W, full-spectrum) |
C2H5OH |
70.00 μmolg−1 h−1 |
/ |
[79] |
ZIF-67@PMO10V2 |
Xe Lamp (300 W, full-spectrum) |
C2H5OH |
74.80 μmolg−1 h−1 |
/ |
[79] |
ZIF-67@PMO9V3 |
Xe Lamp (300 W, full-spectrum) |
C2H5OH |
134.60 μmolg−1 h−1 |
/ |
[79] |
ZIF-67@PMO4V8 |
Xe Lamp (300 W, full-spectrum) |
C2H5OH |
149.00 μmolg−1 h−1 |
/ |
[79] |
Au@UiO-66 |
Xe Lamp (300 W, L42) |
None |
18.90 μmolg−1 h−1 |
1.54 (520 nm) |
[80] |
MOF-74@C3N4 |
Xe Lamp (300 W, L40) |
CH3OH |
330.00 μmolg−1 h−1 |
/ |
[81] |
MIL-125@TiO2 |
Xe Lamp (300 W, 200 mWcm−2) |
None |
102.70 μmolg−1 h−1 |
/ |
[82] |
GSCe (Graphene@Ce-UiO-66) |
LED (6 W, 365 nm) |
None |
110.24 μmolg−1 h−1 |
9.25 (365 nm) |
[83] |
9MX-MOF |
Xe Lamp (300 W, full-spectrum) |
Na2SO3 |
88.79 μmolg−1 h−1 |
/ |
[84] |
MOF derivatives |
Ru–In2O3 HPNs |
Xe Lamp (300 W, AM 1.5 G filter) |
CH3OH |
44.50 μmolg−1 h−1 |
/ |
[85] |
2.1. Pristine MOFs
2.1.1. Transition Metal-Based MOFs
The unoccupied and occupied d-orbitals in some transition metals-based MOFs have appropriate energy and symmetry, which makes these MOFs effectively adsorb and activate N
2. The empty
d orbital of open metal sites in MOFs can accept the electrons from the occupied
σ orbital of N
2. At the same time, the occupied
d orbitals of open metal sites donate electrons to the empty
π* orbital of N
2. The back donated bonds not only weaken N≡N, but also strengthen the metal-nitrogen bond
[86], making transition metals-based MOFs for photocatalytic nitrogen fixation possible.
Cerium-Based MOFs
Cerium, with its electron configuration of [Xe]4
f26
s2, exhibits flexible valence transformation behavior between Ce
3+ and Ce
4+, with occupied 4
f1 and unoccupied 4
f0 orbitals, respectively. On this basis, the cerium with empty orbits and filled orbitals can mimic
π back donation for further catalysis
[87].
Recently, Zhang et al. applied MOF-76(Ce) to effectively transform N
2 into NH
3, mimicking π back donation
[75]. MOF-76(Ce) nanorods with Ce coordinate unsaturated sites (Ce-CUS) were prepared using the solvothermal method (
Figure 2a). Under full-spectrum light source irradiation, the photogenerated electrons were first transferred to Ce-CUS sites, then to the π antibonding orbital of adsorbed N
2 molecules on it. The electrons entering the π antibonding orbital would weaken the N≡N bond, significantly improving photocatalytic nitrogen fixation efficiency. The photocatalytic nitrogen fixation stability of MOF-76(Ce) is comparable to CeO
2 (
Figure 2b). Moreover, due to the synergy between Ce-CUS and N
2, the photocatalytic nitrogen fixation activity of MOF-76(Ce) is higher than CeO
2, with an activity of 34.2 μmolg
−1 h
−1. It should be noted that this is the first kind of study that involved using MOFs for nitrogen fixation.
Figure 2. (
a) Synthesis diagram of MOFs-76(Ce). (
b) MOFs-76(Ce) stability test of photocatalytic nitrogen fixation. Reproduced with permission
[75]. Copyright 2019, American Chemical Society.
Titanium Metal-Based MOFs
Ti-based MOFs have been widely explored in photocatalytic hydrogen production
[88][89][90], photocatalytic CO
2 reduction
[91][92], and environmental protection; however, photocatalytic nitrogen fixation is still in its preliminary stages of development.
Recently, the group initially synthesized three functional group-decorated isostructural MOFs (NH
2-MIL-125 (Ti), OH-MIL-125 (Ti), and CH
3-MIL-125 (Ti)) for visible-light-driven photocatalytic nitrogen fixation
[69]. The introduced functional groups effectively enlarged the light-harvesting range of MIL-125 (Ti) from the ultraviolet (UV) region to the visible light region. After being exposed to visible light (400–800 nm) for about 15 h, the produced ammonia of NH
2-MIL-125 (Ti), CH
3-MIL-125 (Ti), and OH-MIL-125 (Ti) reached 183.76 μmolg
−1, 20.88 μmolg
−1, and 75.48 μmolg
−1, respectively. It should be noted that no sacrificial agent participated in the photocatalytic nitrogen fixation process for those MOFs. The reason for the highest nitrogen fixation rate of NH
2-MIL-125 (Ti) can be explained by the introduction of NH
2, which significantly increased light absorption to 550 nm, and by the exposed Ti coordinational unsaturated sites induced by a linker defect. The photocatalysis mechanism can be illustrated as follows: under visible light irradiation, photogenerated electrons in organic ligands are transferred to exposed Ti coordinational unsaturated sites and reduce Ti
4+ to Ti
3+; then, the electron in Ti
3+ is further transferred to the π anti-bond of N
2 to weaken the strong N≡N bond, eventually reducing the N
2 to NH
3.
Zirconium-Based MOFs
Defective UiO-66(Zr) series MOFs reflect excellent application prospects in photocatalytic hydrogen production, pollutant degradation, and absorption, which result from their large surface areas and suitable pore structures
[93][94][95].
Recently, UiO-66-UV-vis with linker defects induced by light was published by Gao et al.
[70] to improve photocatalytic nitrogen fixation activity. In order to improve the increase in photocatalytic nitrogen fixation activity that was attributed to linker defect but not cluster defect, three different types of UiO-66, UiO-66-fresh, UiO-66-UV-vis, and UiO-66-PSE, were studied for photocatalytic nitrogen fixation. UiO-66-fresh, UiO-66-UV-vis, and UiO-66-PSE stand for fresh prepared UiO-66, UV-vis light treated UiO-66, and defect repaired UiO-66 through a post-synthetic ligand exchange process (PSE), respectively. Compared with UiO-66-fresh, the coordinated formic acid and acetic acid were removed in UiO-66-UV-vis after exposure to ultraviolet light. The exposed coordination of unsaturated metal sites greatly enhanced the activity of UiO-66-UV-vis up to 256.6 μmolg
−1 h
−1. The coordination unsaturated Zr node on UiO-66 can inject photogenerated electrons into the antibonding π-orbitals of N
2 to promote the activation and dissociation of N
2. In contrast, the exposed coordination unsaturated metal sites were recovered by the terephthalic acid linker in UiO-66-PSE, which resulted in lower photocatalytic activity, even lower than that of UiO-66-fresh.
Guo et al. also introduced defect Zr-based MOFs created by thermal treatment, using UiO-66(SH)
2-200 as the photocatalyst for the reduction of N
2 [71]. The Zr clusters were dehydrated by thermal treatment, thus providing accessible [Zr
6O
6] sites for N
2 adsorption and activation. The optical temperature was 200 °C. SH groups were also introduced into UiO-66 to improve the absorption edge of the light to visible light. UiO-66(SH)
2-200 shows a photocatalytic nitrogen fixation activity up to 32.4 μmolg
−1 h
−1 under visible light. In-situ DRIFTS (diffuse reflectance infrared Fourier transform spectroscopy) revealed that the N
2 molecule was gradually reduced to an N
xH
y intermediate and to NH
3, finally
[96]. DFT (density functional theory) calculations further revealed that the photoelectron initiates the reduction of the N
2, immediately followed by the protonation of the activated N
2.
Iron-Based MOFs
Fe is an essential transition metal in nitrogenase, which plays a vital role in photocatalytic nitrogen fixation. There have been many types of research on reducing N
2 by utilizing the Fe active site
[97][98][99][100]. When the Fe metal site is exposed for adsorbing N
2, the unpaired electrons of the
d orbital in Fe will transfer to the
π antibonding orbital of the N
2 molecule to form a strong Fe–N bond that weakens the N≡N bond.
Recently, in order to prove the importance of the transition metal Fe for photocatalytic nitrogen fixation
[72], Li et al. studied the nitrogen fixation performance of MIL-101(Fe), MIL-100(Fe), MIL-88(Fe), and MIL-101(Cr). Notably, MIL-101(Fe) exhibited the highest photocatalytic nitrogen activity (50.36 μmolg
−1 h
−1), whereas isostructural MIL-101(Cr) had almost no activity. DFT calculations revealed that MIL-101(Fe) showed more electronic supply capacity, higher adsorption energy of N
2, and a lower reaction barrier than MIL-101(Cr), confirming the important role Fe has during photocatalytic nitrogen fixation .
Owing to its unique multi-iron metallocluster (Fe
2+3Fe
3+4M
3+, M = Mo, V, Fe), nitrogenase exhibits excellent nitrogen fixation activity. Recently, Zhao et al. synthesized a MIL-53(Fe
2+/Fe
3+) containing both Fe
2+ and Fe
3+ for photocatalytic nitrogen fixation
[73]. In this MOF, Fe
2+ and Fe
3+ simulated Fe
2+ activity sites and high valence metal ions (M = Mo, V, Fe) in nitrogenase. The Fe
3+ in MIL-53(Fe
2+/Fe
3+) can be partly reduced into Fe
2+ by ethylene glycol (EG), and the Fe
2+/Fe
3+ ratio can be regulated from 0.18:1 to 1.21:1 by changing the EG content. Notably, when the ratio of Fe
2+/Fe
3+ was 1.06:1, photocatalytic nitrogen fixation activity reached its highest value of 306 μmolh
−1 g
−1.
The proposed mechanism for photocatalytic nitrogen fixation by MIL-53(Fe2+/Fe3+) can be divided into six steps. (i) N2 adsorbs onto Fe2+ active sites of MOF, in which the electrons transfer to N2 to form the Fe3+-azo intermediate. (ii) Then, the hydrogen transfer quickly occurs in the Fe3+-azo intermediate. (iii) In the photo-excitation stage, holes transfer to the Fe–O− radical, and Fe3+ reduces to Fe2+ by electrons. (iv) The sacrificial agent K2SO3 eliminates the holes, and Fe2+-hydrazo transforms into Fe3+-hydrazo, with H2N–NH2 species appearing. (v) Fe3+-hydrazo transfers to Fe3+-amine with further hydrogenation. (vi) Finally, the NH3 releases gradually, benefitting from continuous hydrogenation.
Transitional Bimetallic MOFs
Similarly to MIL-53(Fe
2+/Fe
3+), An et al. developed bimetallic MOFs containing Zr and Hf for photocatalytic nitrogen fixation. Zr simulates the Fe
2+ active site in nitrogenase, acting as the active site through the π antibonding mechanism. Hf imitates the high valence metal in nitrogenase to promote electron transfer and utilization. An SH group was also introduced to extend the absorption edge to the visible light region
[77]. As the SH group expands the absorption spectrum to 502 nm and the synergistic effect of Zr and Hf, U(0.5Hf)-2SH with 50% Hf exhibits extremely superior photocatalytic nitrogen fixation activity (116.1 μmolh
−1 g
−1) under visible light. The constructed ligand-to-metal-to-metal electron transfer (LMMET) pathway in MOFs promotes the transferring and utilization of photogenerated electrons. Under visible light irradiation, the electrons move from the highest occupied molecular orbit (HOMO) to the lowest occupied molecular orbit (LOMO). At the same time, the holes are consumed by the sacrificial agent K
2SO
3, the electrons are transferred to the multi-metal clusters, and finally the N
2 is reduced to NH
3. Except for zirconium- and hafnium-based MOFs, An et al. further synthesized a stable amino-functionalized UiO-66 with bimetallic Ce-Hf nodes for photocatalytic nitrogen fixation
[78]. In this MOF, the introduced NH
2 group expands the absorption edge, the Ce species acts as an electron buffer tank to enhance electron transfer, and the Hf species plays the part of active catalytic sites to improve the selectivity of the nitrogen fixation reaction. When the molar ratio of Ce-Hf is 1:1, the nitrogen fixation activity was the highest (158.4 μmolh
−1 g
−1) under visible light, with K
2SO
3 as the sacrificial agent.
2.1.2. Post-Transition Metal-Based MOFs
Compared with transition metal-based MOFs, the post-transition metal-based variations are rarely reported to reduce nitrogen to ammonia as photocatalysts.
Gadolinium-Based MOFs
Hu et al. developed two viologen-based radical-containing metal–organic frameworks, Gd-IHEP-7 and Gd-IHEP-8
[76]. A single-crystal-to-single-crystal (SCSC) transformation occurred from two-dimensional (2D) Gd-IHEP-7 to three-dimensional (3D) Gd-IHEP-8 when heating the Gd-IHEP-7 in the air at 120 °C. With a rearrangement of the Gd
3+ coordination environment, enhanced photocatalytic nitrogen fixation activity emerged with the SCSC transformation. Chemisorption of N
2 onto the catalytic sites is a pre-condition for photocatalytic nitrogen fixation. Both the adsorbed N
2 on active Gd metal sites for Gd-IHEP-7 and Gd-IHEP-8 were activated, evidenced by the elongated N–N bond length and the shortened Ga–N bond length. Compared with the distal (D) route, the alternative (A) route is more favorable for both Gd-IHEP-7 and Gd-IHEP-8. While even theoretical calculations indicate that similar photocatalytic nitrogen fixation pathways exist for both RMOF (Gd-IHEP-7, Gd-IHEP-8), the intermediates for Gd-IHEP-8 showed better stability, resulting in a better nitrogen fixation activity of 220 µmolh
−1 g
−1.
Aluminium-Based MOFs
The post-transition metals can not only function as active sites as in Gd-IHEP-8, but they also act as metal nodes that impart high framework stability to MOFs. Recently, Shang et al. developed two porphyrin-based metal–organic frameworks for photocatalytic nitrogen fixation, named Al-PMOF and Al-PMOF(Fe). Compared with Al-PMOF, Al-PMOF(Fe) not only has Al as the metal node to stabilize the framework of the MOF, but also has Fe as the active center to adsorb and reduce the N
2 to NH
3 [74]. The structure of Al-PMOF(Fe) is shown in
Figure 3a. The atomically isolated Fe in Al-PMOF(Fe) was proven through X-ray absorption spectroscopy (XAS) and X-ray photoelectron spectroscopy (XPS). Photocatalytic nitrogen fixation experiments showed that Al-PMOF(Fe) had better activity than Al-PMOF under visible light, and that the produced NH
3 originated from N
2. It was confirmed that the addition of Fe as the active center effectively increased the adsorption of N
2 and further enhanced the photocatalytic performance (
Figure 3b,c). DFT calculations were further applied to establish the photocatalytic nitrogen fixation reaction pathway (
Figure 3d–f). The first hydrogenation from N
2* to N
2H* showed no obvious difference between alternating and distal pathways, but further hydrogenation showed that the alternating pathway seemed more likely to occur. Notably, the release of 1NH
3 in the distal process requires significant energy, making the reaction difficult.
Figure 3. (
a) The structure of Al-PMOF(Fe). (
b) N
2 adsorption isotherms. (
c) Photocatalytic nitrogen fixation activity of Al-PMOF(Fe) and Al-PMOF. (
d) Charge different maps for Al-PMOF(Fe) adsorbing N
2. (
e) Alternating and (
f) distal pathways in Al-PMOF(Fe). Reproduced with permission
[74]. Copyright 2021, American Chemical Society.
MOFs can also function as photosensitizers and transfer the photogenerated electrons to active sites in other functional materials that are in contact with them. For example, Ding et al. combined N-defect thin film g-C3N4 (DF-C3N4) with nano MOF to form Nano MOF-74@DF-C3N4 composite for enhanced photocatalytic nitrogen reduction. A Z-type heterojunction was formed between Nano-MOF-74 and DF-C3N4, which clearly improved the separation efficiency of photogenerated electrons and holes. The Z-type heterojunction photocatalyst structure first proposed by Bard et al. in 1979, can provide the entire system with a stronger redox ability to promote photocatalytic activity. It should be noted that another carrier transfer process of type II is competitive with the Z-type process, and the fluorescence lifetime results indicated that the Z-type is the main process. In this Z-type carrier transfer process, the photogenerated electrons in the conduction band of MOFs are firstly transferred to the valence band of DF-C3N4, then become re-excited to the conduction band of DF-C3N4, and finally anticipate the reduction of N2 adsorbed on N defect sites of DF-C3N4.
Another similar example is the MOFs composite MIL-125@TiO2 with core-shell structure synthesized by Wang et al., using the post thermal solvent method (Figure 10a) [95]. The thickness of TiO2 nanosheets can be controlled by the reaction time with thioacetamide. Under the synergistic effect of MIL-125 and TiO2, MIL-125@TiO2-2 h exhibited an activity up to 89.5 µmolg−1 h−1 under visible light, which was much higher than the pristine MIL-125 (Figure 10b). In MIL-125@TiO2, photogenerated electrons can be transferred to both the coordination unsaturated Ti sites of defect MIL-125 and TiO2, and further reduce the adsorbed N2 to NH3.
MXene is a new type of two-dimensional material with a graphene-like structure. It has the advantages of a large specific surface area, excellent conductivity, and abundant surface groups. Qin et al. synthesized MXene/MOF composites (Ti
3C
2-QD/Ni-MOF, formed as type II heterojunction; QD stands for quantum dot) by combining MXene QDs and Ni-MOF through self-assembly, which improved the photocatalytic nitrogen fixation efficiency to 88.79 μmol g
−1 h
−1 [97]. Under light irrigation, the electrons and holes in Ti
3C
2-QD were separated. Then, the photo-excited electrons were transferred to the conduction band (CB) of Ni-MOF to participate in the photocatalytic nitrogen fixation reaction. In this system, the heterojunction formed by Ti
3C
2-QD and Ni-MOF effectively promotes the separation of electrons and holes. Ni effectively adsorbed N
2 as an active site, and promoted the nitrogen fixation process.
MOFs can also function as porous scaffolds to support functional units, and enhance the mass transfer of N
2 to produce NH
3. For example, Chen et al. used the UiO-66 membrane as a nanoreactor to support gold nanoparticles (AuNPs)
[93] and realized a direct plasma photocatalytic nitrogen reduction reaction at room temperature and ordinary pressure. Notably, in the Au@UiO-66, UiO-66 not only effectively restricts the highly dispersed AuNPs but also ensures effective contact between AuNPs and N
2 molecules in the aqueous solvent. In the gas film solution reaction interface, the N
2 molecule can proceed straight into the Au@UiO-66 membrane. Each AuNP in UiO-66 could not only generate electrons through photo-excitation, but also promote the reduction of N
2 as a co-catalyst. (
Figure 4a). The mass loading of gold was the critical factor that affected photocatalytic nitrogen fixation. When the loading amount of Au is 1.9 wt %, the conversion of nitrogen to ammonia reaches its highest level of 0.14 mmol g
−1 h
−1 (
Figure 4b).
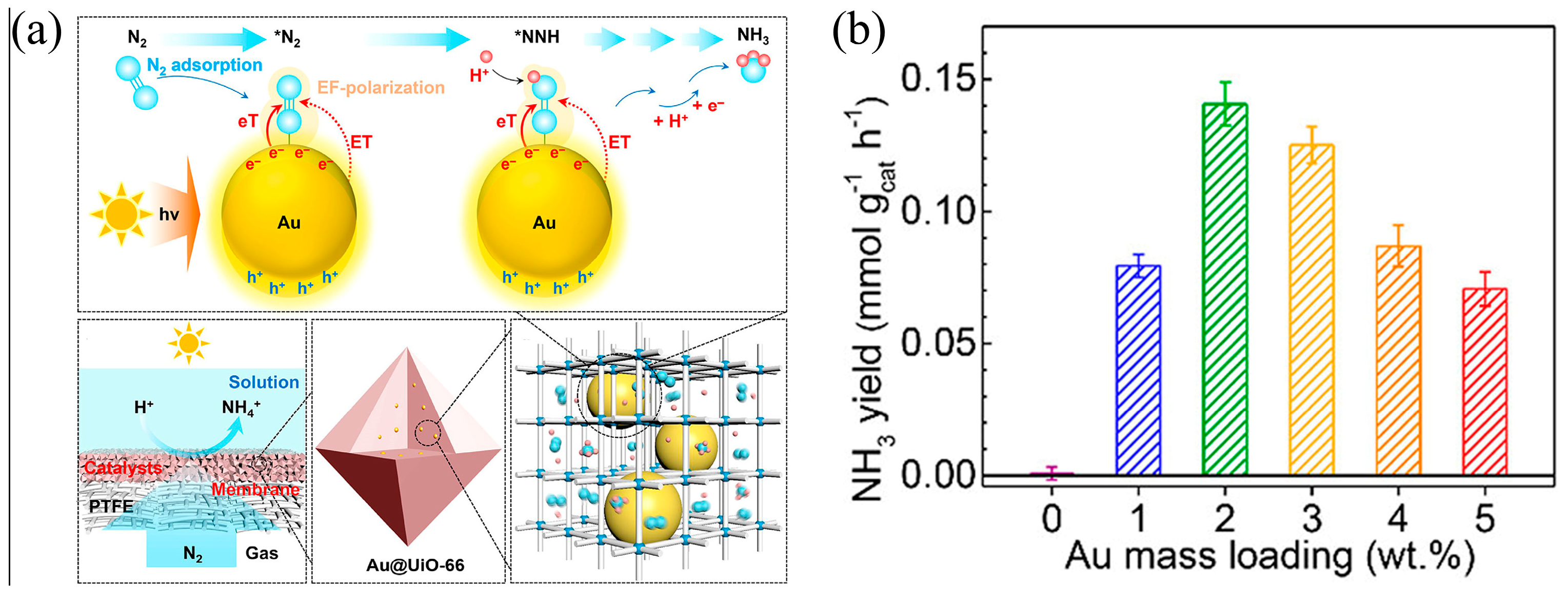
Figure 4. (
a) Schematic diagram of direct plasma photocatalytic nitrogen reduction on AuNP encapsulated by UiO-66 matrix. (
b) Direct plasma photocatalytic nitrogen reduction performances in the PiS system. Reproduced with permission
[93]. Copyright 2021, American Chemical Society.