1. Structural Features and Physicochemical Properties of Stoichiometric and Non-Stoichiometric Titanium Oxides
TiO
2 belongs to n-type semiconducting materials
[1]. Many TiO
2-based heterostructures are used in the design of sensors
[2][3] and biosensors
[4][5]. However, all the most popular forms of titanium oxides are characterized by specific bandgaps, which are as follows: (i) anatase by 3.02 eV (ii) rutile by 3.23 eV; (iii) brookite by 2.96 eV
[6]. The annealing procedure is mostly used for the conversion of one titanium oxide phase into another one. Besides stoichiometric titanium oxide, plenty of non-stoichiometric forms were identified, among them very attractive conducting/semiconducting characteristics and gas-sensing properties
[3]. They have Magnéli phases, which are described by Ti
nO
2n−1 stoichiometry, where n = 4, …, 10. Their Magnéli phase neighbor is titanium pentoxide (Ti
3O
5), where n = 3 with a stoichiometry of Ti
nO
2n−1, which appears in a variety of different forms (that are indicated as α−, β−, γ−, δ−, and λ−)
[7]. Stoichiometry of titanium pentoxide corresponds to that of the Magnéli phases (Ti
nO
2n−1). Titanium pentoxide forms monoclinic crystals with the following lattice constants: a = 9.9701 Å, b = 5.0747 Å, c = 7.1810 Å, β = 109.865°. Moreover, titanium pentoxide, as well as some other Magnéli phases (e.g., Ti
4O
7), exhibit superconductivity when cooled down below 7 K temperature
[8].
The most significant difference between titanium pentoxide and the Magnéli phases is determined by their different crystal structures. Magnéli phases contains shear planes based on TiO
2(rutile) [9], while in titanium pentoxide, such planes are absent
[3][8]. A temperature of 400 °C is optimal for the appearance of TiO
2(anatase) intergrowths within Ti
3O
5 crystals based on TiO
2−x/TiO
2-based heterostructures
[10]. The incorporation of intergrowths based on TiO
2(anatase) in the structure of titanium pentoxide (Ti
3O
5) improves their conductivity and some photoluminescence-related characteristics
[3]. It should be noted that such non-stoichiometric titanium oxides can be spontaneously oxidized for this reason and significant attention should be paid to the stabilization of these structures during their usage in the development of gas sensors. It should be noted that at different oxidation states, titanium oxides have different crystal structures
[11][12], which starts from rutile for TiO
2 and anatase for Ti
10O
19 and then it turns into the triclinic structure for many stoichiometries, ranging from Ti
9O
17 until Ti
4O
7, monoclinic for γTi
3O
5, tetragonal for Ti
2O
3 and hexagonal for TiO, Ti
2O and metallic titanium.
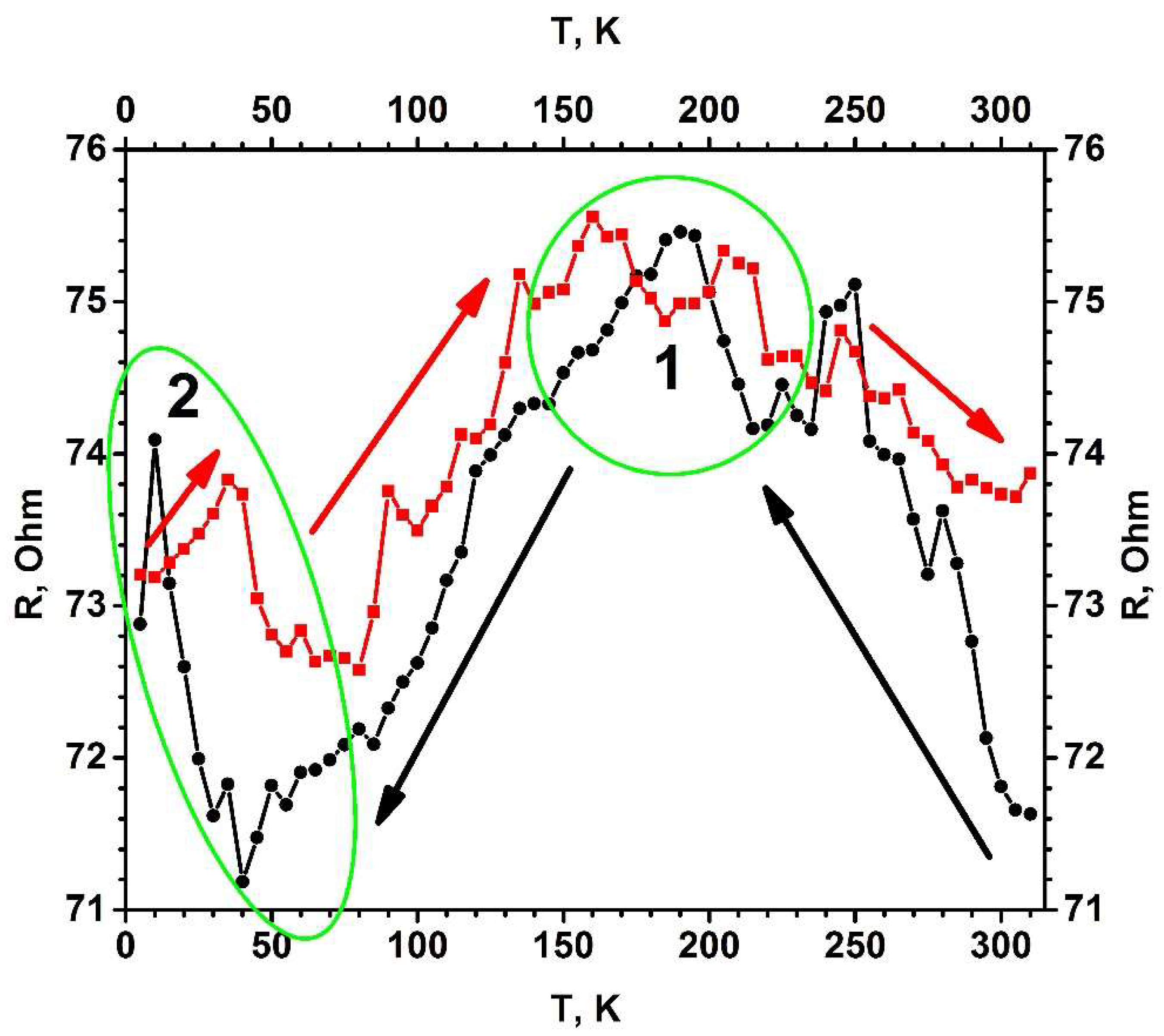
Figure 1. Temperature dependence of electrical resistance (R(T)) for the TiO2−x/TiO2(400 °C)-based hetero-structure. Temperature was changed in two ways (indicated by black and red arrows): (i) black cycles shows points measured by cooling down, (ii) red squares shows points by increasing temperature. Measurements were performed in vacuum using helium cryostat. Figure adapted from [3].
The electrical conductivity of most non-stoichiometric titanium oxides is significantly higher than the conductivity of any allotropic form of stoichiometric titanium oxides (TiO
2). The most significant increase in sensitivity is observed for titanium oxides with Ti
nO
2n−1 stoichiometry, when index ‘n’ is in the range of 4–10
[13]. Some compounds with the above-mentioned stoichiometry form Magnéli phases, which are characterized by metallic conductivity and even by superconductivity
[14].
Non-stoichiometric titanium oxide-based layers based on Magnéli phases have well conducting intergrowths based on Ti
nO
2n−1 planar moieties that penetrate the TiO
2-based matrix
[3]. Non-stoichiometric titanium oxides, such as Ti
2O
3 and/or Ti
3O
5, which do not form real Magnéli phases anyway, are much better at conducting in comparison to stoichiometric TiO
2 [3][8]. These n-type semiconducting titanium oxides have a high concentration of ‘oxygen vacancies’, which are responsible for the mobility of electrons through their structure
[15] and baseline resistance
[16]. It should be outlined that these ‘oxygen vacancies’ are also responsible for the sensitivity towards both oxidizing and reducing gases
[3]. During the design of the sensing layer, initially formed stoichiometric titanium oxide-based layers can be chemically reduced into non-stoichiometric titanium oxide (TiO
2−x) and/or Magnéli phases
[17]. Magnéli phases are observed when the oxygen concentration in TiO
2−x structure is decreased and ‘x’ value is between 0.1 and 0.34
[3][18]. Non-stoichiometric titanium oxide structures containing Magnéli phases are chemically stable and rather well conducting. For these reasons, they are often applied in wastewater treatment and the design of batteries and fuel cells
[19][20]; the same characteristics are required for gas sensors.
Non-stoichiometric titanium oxide-based structures can be developed using several approaches, namely plasma-treatment
[21], laser irradiation-based modification
[22], reduction by metallic zinc
[23], bombardment by high-energy particles
[24] and thermo-chemical approaches
[12]. However, the formation of large Ti
3O
5 monocrystals is rather challenging because titanium oxides are polymorphic
[8]. In some situations, stoichiometric titanium oxide can be easily turned into a non-stoichiometric one by suitable doping and/or reduction.
During the formation of TiO
2−x/TiO
2-based heterostructures, the ratio between stoichiometric titanium oxide and non-stoichiometric titanium oxide can be significantly increased by thermal treatment in reducing the gas atmosphere
[25]. In several works, the transition between the insulator state and metallic state of Ti
3O
5 (β and λ forms of Ti
3O
5, respectively) was performed by pulses of visible light
[7] and by thermal treatment, which induced the conversion of α form into β form at 450 K
[26] and the conversion of δ form into γ form at 240 K
[7]. The phase transition of Ti
3O
5 at 240–450 K is the most important for the adjustment of conductivity of this semiconducting material-based layers, e.g., the switching between metal and insulator states was observed at 350 K
[8].
In some situations, non-stoichiometric titanium oxide can be formed by the partial oxidation of metallic titanium layers, which is followed by thermal treatment and annealing
[3]. Sensors based on such structures, which are differently thermally treated and annealed, can be used in the formation of sensors with very different selectivity and sensitivity, which are suitable for the development of sensor arrays. Non-stoichiometric titanium oxide-based sensors. despite some significant advantages related to better catalytic activity and conductivity, have some disadvantages in comparison to those based on stoichiometric titanium oxides related to their insufficient stability at ambient conditions. In addition, it should be noted that the selectivity of these sensors is not superior.
2. Pristine Titanium Oxide-Based Gas Sensors and Their Sensing Mechanisms
Stoichiometric TiO
2-based gas sensors show high sensitivity to different gases. It should be noted that TiO
2-based gas sensors can rely on several different sensing mechanisms, which differ the most significantly for the determination of reducing gaseous compounds, such as H
2, H
2S, NH
3, CO, CH
3OH, C
2H
5OH, etc. and for oxidizing gaseous compounds, such as O
2, NO
2, CO
2 [27][28][29]. The changes in electrical resistivity of the TiO
2-based layer are mostly used for the determination of analytical signals; therefore, the assessment of analytical signals generated by such sensors is rather simple. In some situations, measurement protocols were advanced by the determination of photoluminescence signals
[3][17][30], which are generated by semiconducting TiO
2 structures
[31]. Some above-mentioned photocatalytic and photovoltaic properties can be improved by laser-based treatment
[32]. However, the main disadvantage of TiO
2-based sensors is poor selectivity towards gaseous materials, which significantly complicates the application of these analytical devices. Therefore, in order to improve selectivity, various heterostructures containing TiO
2 hybridized with many other semiconductors have been developed
[33], e.g., the research group has developed a TiO
2−x/TiO
2-based self-heating heterostructure for the determination of NH
3, CH
3OH and C
2H
5OH
[3].
Table 1. Characteristics of titanium oxide-based sensors [34].
It was also reported that TiO2 combined with La0.8Sr0.2Co0.5Ni0.5O3 perovskite was applied for the determination of CO [44], TiO2/V2O5-based heterostructure for ozone [45], TiO2/SnO2-based heterostructure for NO2 and CO [46][47]; TiO2/graphene (TiO2/GO)-based heterostructure for toluene determination at 298 K [48]. The action of TiO2/GO-based heterostructures based on the formed n-n hetero-junction is enhanced by UV irradiation and is addressed in the band diagram. The bandgap of the hetero-junction in the TiO2/GO-based heterostructure is 4.7 eV [49] which is higher than that of 4.4 eV and is usually observed for GO-based structures [50]; the formed hetero-structure has both accumulation and depletion layers [51]. In this structure, a Schottky barrier is formed; therefore, the depletion layer in TiO2 is thicker, and the number of electrons in the GO interface increases. The interaction of the adsorbed gases with the oxidized functional groups of GO induces variation in the resistance of the TiO2/GO-based heterostructure, which forms a sensing layer. In this heterostructure, TiO2 is contributing by increasing the number of active sites suitable for the adsorption of gas molecules [52]. In some particular situations, activation energy can be lowered by adsorbed gas molecules, which increases the catalytic activity of the TiO2 layer [53]. This property increases the sensitivity towards some reducing gases, such as ammonia and so on.; however, the active sites of GO are very sensitive towards moisture that increases electrical resistance, due to the interaction of water molecules with oxygen-based (carboxyl, carbonyl and hydroxyl) functional groups [54]. UV irradiation enables to solve this disadvantage by the increase in the depletion layer in TiO2 and enhancement of the accumulation layer in GO. Due to this reason, the electrons are shifted towards GO and reduced carboxyl, carbonyl and hydroxyl groups, which become unsuitable for the adsorption of water molecules [55]. This reduced GO establishes a p-n junction with TiO2, which at the interface is characterized by decreased junction width. UV irradiation of TiO2 induces the ‘injection’ of electrons into GO and in such a way that electron–hole pair recombination is prevented [56]. The surface concentration of adsorbed oxygen molecules decreases, which also reduces water sorption to this heterostructure [57]. The number of carboxyl, carbonyl and hydroxyl groups in GO structure can be partly restored by the UV irradiation-induced action of TiO2 [58].
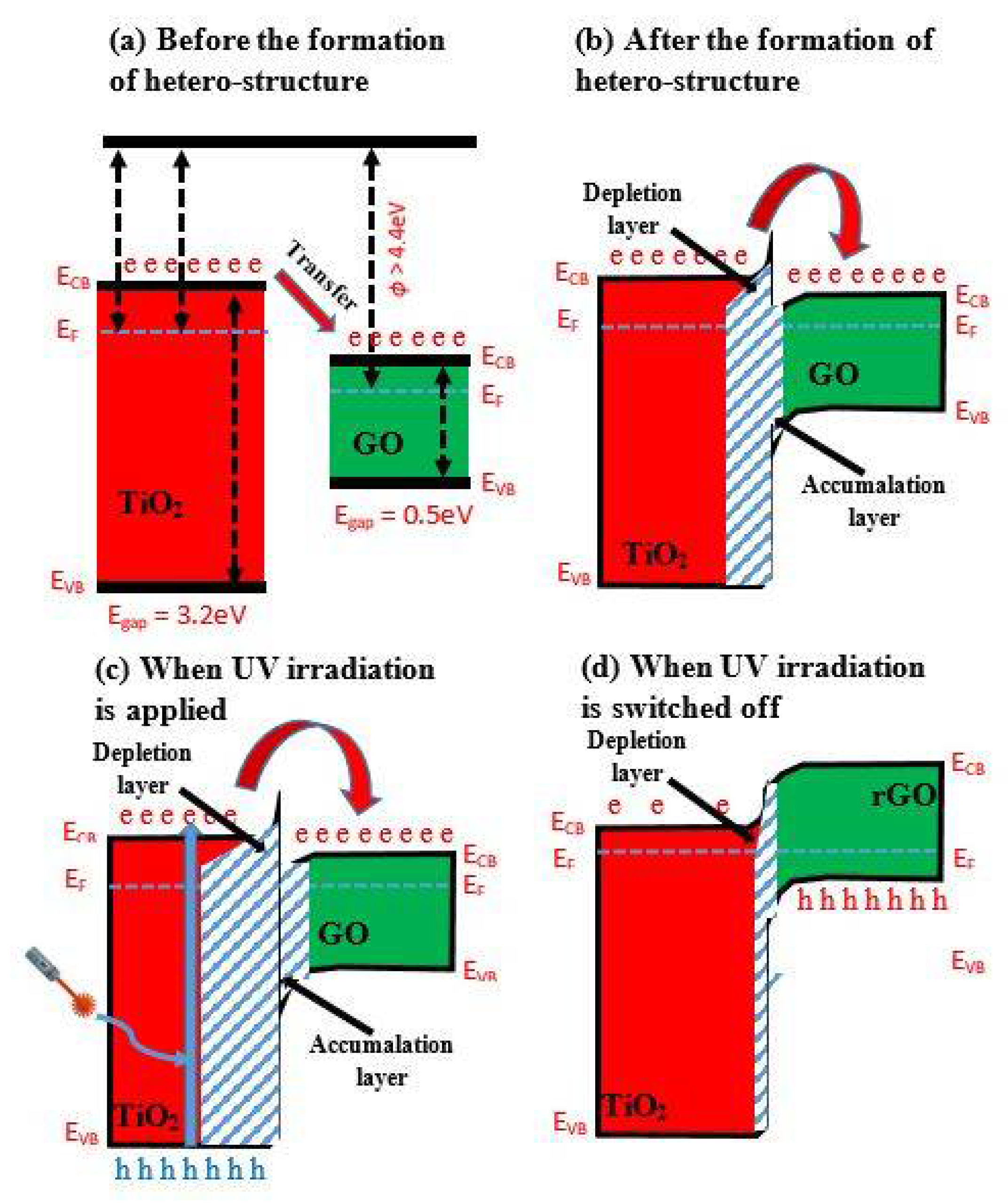
Figure 2. Band diagram of TiO2/GO hetero-structure (a) before the formation of hetero-structure, (b) after the formation of hetero-structure, (c) when UV irradiation is applied, and (d) when UV irradiation is switched off. (‘e’ is an electron; ‘h’ is a hole) [34].
The heterostructure based on TiO
2/SnO
2 is also very interesting because the depletion of energetic layers in the molecular orbitals of TiO
2 electrons is induced when TiO
2 is connected to SnO
2 [46]. It should be noted that nanoparticle-based structures are preferable for the development of gas sensors
[59] because the radius of nanoparticles based on some semiconducting materials that are used in the design of gas-sensors are in the same range as the Exciton Bohr radius; for this reason, such particles are very suitable for the design of gas sensors
[60].
SnO
2 has great charge-carrier mobility, which is the most important factor for gas sensors based on resistivity measurements
[47]. SnO
2-based gas-sensing layers are cheap and stable at ambient conditions
[61] however, it should be taken into account that sensors based on stoichiometric TiO
2 and SnO
2 operate at a rather high temperature, which exceeds several hundred degrees
[60]. However, such sensors consume a lot of energy for the heating of the sensing layer; therefore, due to energy saving related issues, sensing layers capable to operate at low temperatures are under very special interest, e.g., sensing layers based on Au/SnO
2 core-shell structures can operate in the temperature interval of 25–80 °C
[62]. The investigations of non-stoichiometric titanium oxide-based sensors shows that these sensors can operate even at room temperature
[3][17]. It is expected that heterostructures based on SnO
2/TiO
2 can be applied in low temperature gas sensors; therefore, some nanostructures were designed, which are as follows: TiO
2 nanobelts covered by SnO
2 [63], TiO
2/SnO
2-based core-shell nanofibers
[64], SnO
2 layers doped by TiO
2 [65], structures based on SnO
2/TiO
2 laminates
[66], SnO
2 nanoparticles wrapped within TiO
2-based nanofibers
[46], SnO
2-based quantum dots formed on a surface of TiO
2 layer were reported to be sensitive towards NO
2 and CO
[67]. Atomic layer deposition (ALD) was used to deposit thin TiO
2 layers and it was demonstrated that tuning of the selectivity can be performed by the adjustment of TiO
2 layer thickness
[68].
Significant energy consumptions for the heating of the sensing layer reduce the applicability of most gas sensors. Therefore, there is a demand for gas-sensing structures that can operate at low temperatures
[69]. The miniaturization of sensing elements is another suitable strategy to reduce energy consumption. Low-temperature sensors based on titanium oxide-based layers were reported for O
2 [66], ozone
[45], formaldehyde
[70], CO
[44], ethanol
[65], C
7H
8 [48], H
2 [71] and other gases
[72].
The ‘self-heating’ of the sensing layer can be achieved when it has rather low resistance and part of electrical energy, which passes through this layer and is converted into thermal energy
[3][17]. However, stoichiometric titanium oxide-based layers are characterised by a high band gap; therefore, the conductivity of these layers is not sufficient for ‘self-heating’ because rather high voltages are required to achieve some effect. On the contrary, this operation mode is very suitable for non-stoichiometric titanium oxide-based sensors, because these layers are good at conducting at low temperatures and in the temperature interval of 72–180 °C, these layers reach very good sensitivity towards some gases
[3][17]. The semiconducting properties and chemical activity of titanium oxide enable sufficient catalytic and photocatalytic activities under UV irradiation to turn into a ‘water splitting’ ability
[32], which eventually can be exploited for sensing purposes. It should be noted that at room temperature (298 K), sensing layers are sensitive to humidity. Therefore, analytical signals generated by adsorbed water molecules interfere with an analytical signal generated by target gases.