Long Coronavirus disease-19 (COVID-19)—and the cognate term “long-haulers”—refers to the persistence of symptoms related to the infection with severe acute respiratory syndrome-coronavirus-2 (SARS-CoV-2)
[1]. This condition is described as persistent and can manifest in various combinations of signs and symptoms in 10%
[2] to 87%
[3] of adults, particularly females, following SARS-CoV-2 infection
[4]. More than 50 long-term effects of SARS-Cov-2 infection have been identified, including fatigue, headache, dyspnea, cognitive impairment—numbness, depression, altered perception of smells and tastes, poor appetite, chronic cough, joint and chest pain, postural orthostatic tachycardia expression of autonomic dysregulation, thermoregulation abnormalities, skin eruptions, and gastrointestinal disorders
[5]. Similar findings have also been reported in children and adolescents
[6]. Recent evidence on vaccination against SARS-Cov-2 suggests that vaccines reduce the risk of long COVID by lowering the chances of contracting COVID-19 in the first place. However, for those who do experience a breakthrough infection, the vaccination might only halve the risk of long COVID—or have no effect on it at all
[7]. Understanding the prevalence of long COVID among vaccinated people has urgent public-health implications as restrictions that limited viral spread are eased in some countries. It could also offer clues about what causes lingering COVID-19 symptoms long after the acute infection has cleared
[7].
2. The Possible Deep Roots of Long COVID-19 and Their Biological Plausibility
Besides genetic predisposition, a diet poor in anti-inflammatory/antioxidant substances with potential immune-modulating and anti-viral activity can be a predisposing but preventable factor for more severe SARS-Cov-2 and very likely also for the development of persistent long symptoms after the acute phase of the disease. This proposition emerged from epidemiologic studies demonstrating that populations with very low death rates were found to have an unusual common feature of eating large quantities of fermented vegetables, including members of the cruciferous and Brassicaceae family
[12]. At the beginning of the pandemic, it was suggested that treatment with a wide range of existing host-directed therapies, including nutrient supplements, was possibly beneficial in the care of 2019-nCoV infection
[13]. Vitamin D deficiency has been associated with an increased number of cases with severity, and with deaths
[14]. In COVID-19 infection, zinc deficiency was found to be linked to higher odds of complications, including deaths
[15], particularly when combined with selenium deficiency
[16], and selenium supplementation has been associated with a better prognosis in those patients
[17]. In addition, low levels of magnesium, which are usually present in all COVID-19 comorbidities
[18], are associated with an increased inflammatory state
[19], while an increased likelihood of survival is seen in severe patients with COVID-19 with higher magnesium blood level on admission to hospital (odds ratio for mortality of 0.032)
[20]. Collectively, these studies suggest that nutritional support may effectively reduce inflammation and oxidative stress, thereby strengthening the immune system during the COVID-19 crisis, and ecological studies have lent support to this suggestion
[12][21][22]. These supplementations may offer additional benefits, providing significant antiviral, anti-inflammatory, antithrombotic, and cytoprotective effects, thus preventing further tissue damage
[23] and favorably modifying the gut microbiome which, in patients with COVID-19, has been found to be concordant with the disease severity and plasma concentrations of several inflammatory cytokines, chemokines, and blood markers of tissue damage, as well as the persistence of symptoms
[24].
3. What Could Be Done for the Prevention
3.1. Vitamins
3.1.1. Vitamin B Group
B group vitamins represent essential micronutrients for energy metabolism, DNA and protein synthesis, and immune cell regulation
[25]. Vitamin B1 influences mitochondrial membrane potential, cytochrome C release, protein kinases, and p38-MAPK; suppresses oxidative stress induced by nuclear factor-kappa b (NF-κB); and has anti-inflammatory properties. Deficiency of vitamin B1 may cause dysfunction of the nervous system; neuroinflammation; T cell infiltration; chemokine CCL2 activation; overexpression of proinflammatory cytokines such as interleukin (IL)-1, tumor necrosis factor (TNF), IL-6, and arachidonic acid products; and induces expression of CD40 by the microglia and CD40L by astrocytes, which provoke the death of neurons
[26]. The active form of vitamin B6, pyridoxal 5′-phosphate (PLP), has consistently been shown to be low in inflammatory conditions and inversely associated with numerous inflammatory markers in clinical and population-based studies, and its low concentration predicts the risk of chronic diseases
[27]. Furthermore, PLP serves as a co-factor in neurotransmitter biosynthesis, as well as a scavenger of reactive oxygen species (ROS)
[28]. Vitamin B12 appears to possess antioxidant properties by scavenging ROS, by the preservation of glutathione, modulation of cytokine and growth factor production and reduction of oxidative stress caused by advanced glycation end-products
[29]. Furthermore, Vitamins B1 (thiamine), B6 (pyridoxine), B12 (cobalamin), and folate play an important role in the pathogenesis of neuropathy and neuropathic pain and on the inflammatory basis of depression
[30]. Pyridoxal-5-phosphate and methylcobalamin are cofactors in peripheral nerve functions and cobalamin facilitates myelinogenesis and nerve regeneration
[31]. Methyl-folate has been shown to improve endothelial function
[32], and the vitamin B group is an essential nutrient for host gut microbiota
[33]. In effect, supplementation of vitamin B12 together with vitamin D and magnesium was shown to prevent severe outcome progression in patients with SARS-Cov2
[34].
3.1.2. Vitamin C
Vitamin C is one of the body’s most important antioxidants and is involved as a co-factor in the synthesis of carnitine; the formation of serotonin, dopamine, and nitric oxide; the synthesis of noradrenaline; the biosynthesis of amidated peptides; hypomethylation of DN; and the degradation of the transcription factor hypoxia-inducible factor 1 alpha implicated in energy metabolism
[35]. Vitamin C contributes to immune defense by supporting various cellular functions of both the innate and adaptive immune system
[35], with a possible preventive effect on autoimmune diseases
[36]. Furthermore, fatigue, pain, cognitive disorders, and depression-like symptoms are known symptoms of vitamin C deficiency
[37] and, although vitamin C plasma levels have not been evaluated in patients with long COVID-19, a deficit is most probable because infections are known to be coupled with high intakes of vitamin C, and insufficiencies in acute infections are frequent
[35]. Indeed, a systematic review of studies evaluating the effect of this vitamin on low energy and weakness suggested that high dose intravenous vitamin C could be a beneficial treatment option in treating fatigue in patients with long COVID-19
[38].
3.1.3. Vitamin D
Vitamin D has an immune-modulating effect and reduces the frequency of infections when taken in daily doses
[39]. The discordant results related to its effect can be attributed to its erroneous use in cumulative monthly doses that are associated with a sudden increase in its plasma levels, followed within a few days by their drastic reduction due to the catabolic effect of the 24-hydroxylase that transforms the vitamin in inactive metabolites
[40]. These continuous oscillations not only neutralize its effect but could also be dangerous in relation to its epigenetic action. Furthermore, this vitamin reduces cellular damage from oxidative stress and stimulates the Nrf2 pathway of signal transduction by promoting the synthesis of anti-inflammatory cytokines (
Figure 1)
[41].
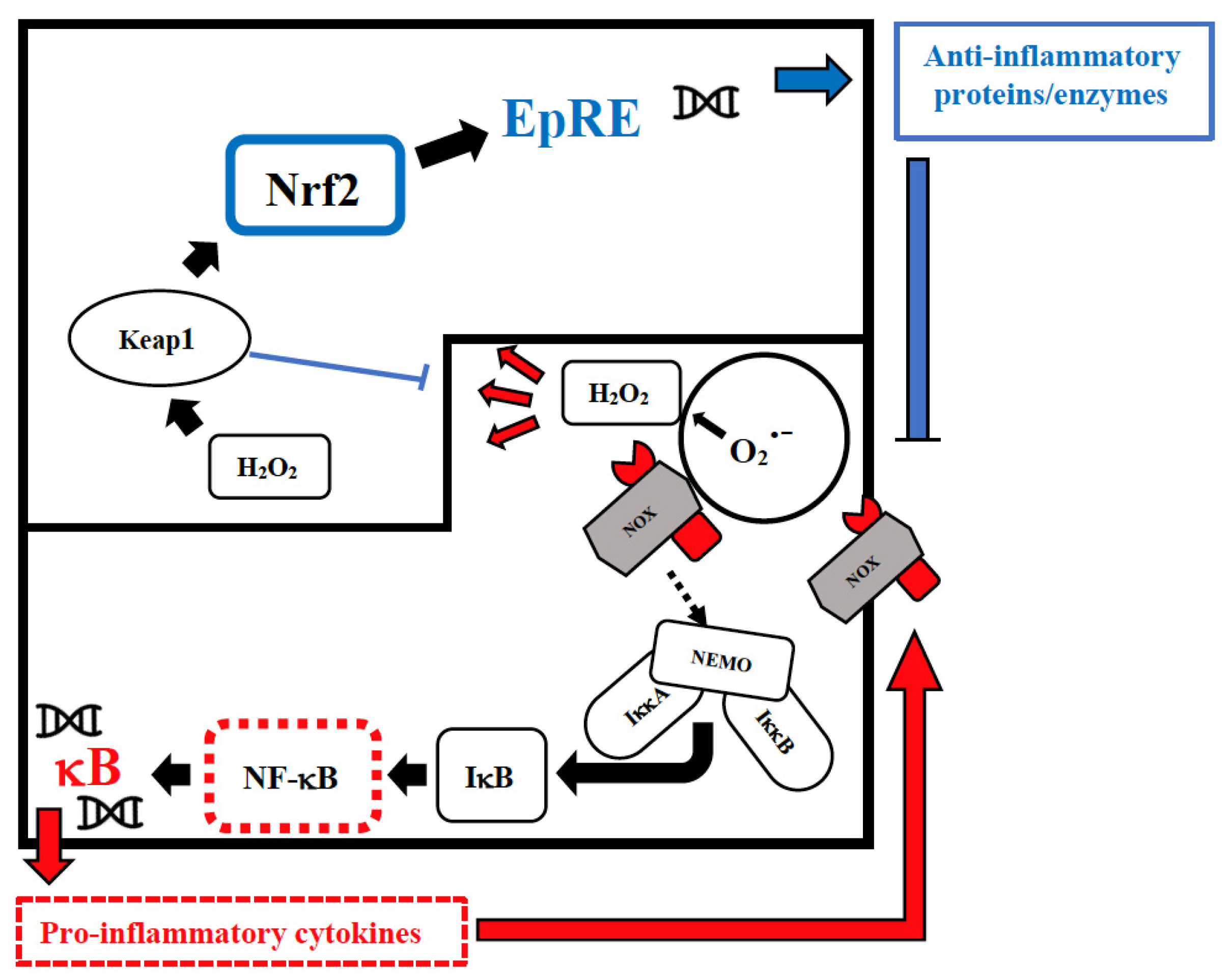
Figure 1. Intranuclear signal transductions can occur in two different pathways: while nuclear factor kappaB (NF-κB) tends to enhance and perpetuate the inflammatory response by triggering the expression of pro-inflammatory cytokines, nuclear factor erythroid 2–related factor 2 (Nrf2) activation through Kelch-like ECH-associated protein-1 (Keap1) oxidation dampens pro-inflammatory signaling by expression of peroxidases and other anti-inflammatory proteins. As E3-ligase, Keap1 also primes inhibitor of NF-κB kinase subunit beta (IKKβ) to degradation via ubiquitination, thereby directly interfering with NF-κB activation. For the sake of clarity, only the reactive oxygen species (ROS)-producing enzyme NADPH oxidase (NOX)-derived H2O2 is shown as an oxidant signal. Depending on the cellular system and the inflammatory stimulus, NOX-derived H2O2 may be supported or replaced by mitochondrial H2O2, lipoxygenase products, and S-alkylating electrophiles derived therefrom. NEMO, NF-κB essential modulator; IκB, Inhibitor of NF-κB.
3.1.4. Vitamin E
Vitamin E (alpha-tocopherol) is a fat-soluble vitamin and a potent antioxidant important in protecting cells from oxidative stress, regulating immune function
[42], maintaining endothelial cell and heart integrity, and balancing coagulation and gut microbiota
[43][44]. It has been demonstrated that vitamin E deficiency impairs the normal functions of the immune system in animals and humans, which can be corrected by vitamin E repletion
[42]. Furthermore, a low level of vitamin E associated with selenium insufficiency results in specific viral mutations, changing relatively benign viruses into virulent ones
[45]. In relation to the long COVID-19 problem, it was reported that a low level of serum alpha-tocopherol improved during the remission phase, as compared to the exacerbation phase, in patients with chronic fatigue syndrome, suggesting that increased oxidative stress may be involved in the pathogenesis and the severity of the symptoms of the syndrome
[46].
3.2. Essential Elements
3.2.1. Magnesium
Magnesium is the most abundant divalent cation in living cells and plays essential roles in the regulation of cell growth, division, and differentiation
[47]. In the heart, magnesium plays a key role in modulating neuronal excitation, intracardiac conduction, and myocardial contraction by regulating several ion transporters, including potassium and calcium channels. Magnesium also has a role in regulating vascular tone, atherogenesis, and thrombosis, and proliferation and migration of endothelial and vascular smooth muscle cells
[48], and it also acts protectively against phosphate-induced kidney injury
[49]. It is involved in numerous biological processes (estimated at over 600) and, when present in physiological concentrations, it controls redox homeostasis, reducing the production of oxygen-derived free radicals in various tissues, lowering inflammation. Mechanisms include its “calcium-channel blocking” effects that lead to downstream suppression of NF-κB, IL-1β, IL-6, and TNF-α, as well as C-reactive protein (CRP) production
[50]. Latent magnesium deficiency is associated with chronic low-grade inflammation
[51], hypertension, metabolic syndrome, type 2 diabetes, and cardiovascular disease
[52], as well as with increased levels of free radicals and mitochondrial dysfunction
[53], possibly causally related to fatigue and myalgic encephalomyelitis/chronic fatigue syndrome
[54], a common manifestation of long COVID-19.
3.2.2. Selenium
Selenium is an essential trace element for mammalian redox biology. Unlike other trace elements that act as cofactors, dietary selenium is converted in the body into aminoacid selenocysteine, which is then incorporated into one of the twenty-five selenoproteins
[55] such as glutathione peroxidase, thioredoxin reductases, and methionine sulfoxide reductase, which are important components of the antioxidant defense systems. Reduced expression of selenoproteins as a result of low/sub-optimal selenium status could alter the molecular pathways involved in stress responses and contribute to an aggressive pro-inflammatory environment due to an imbalance between NF-κB and nuclear factor erythroid 2-related factor 2 (Nrf2) signaling (
Figure 1), which may lead to poorer viral disease prognosis
[56]. On the contrary, selenium supplementation is associated with lower expression of pro-inflammatory NF-κB signaling
[57]. ROS are produced during viral infections, with both positive and negative consequences for the cell
[58]. For example, phagocytic cells produce large amounts of ROS to eliminate a wide variety of pathogens without altering the host cell viability, but ROS have also been found to stimulate viral replication
[59]. This fact is particularly significant for RNA viruses that exhibit the highest known mutation rates, with up to one mutation per genome per generation cycle
[60], and selenium deficiency increases the pathogenicity and severity of infections by benign or mildly virulent strains of Coxsackie and influenza viruses, giving rise to multiple changes in the viral RNA
[61]. Thus, dietary insufficiency of this oligo element impacts not only the immune response of the host, but also the virus itself, and dietary selenium deficiency, which causes oxidative stress in the host, can alter a viral genome so that a normally benign or mildly pathogenic virus becomes highly virulent in the deficient host. This has been shown in animal models for the influenza virus
[62] and human coxsackie enterovirus
[63].
3.2.3. Zinc
Zinc is the second-most abundant trace metal in the human body after iron and an essential component of protein structure and function. It is a vital micronutrient for maintaining cellular physiology
[64]. In fact, it is a structural component of ~750 zinc-finger transcription factors
[65], allowing gene transcription, and it is a catalytic part of approximately 2000 enzymes on all sides of 6 classes (hydrolase, transferase, oxidoreductase, ligase, lyase, and isomerase)
[66]. Zinc acts as a second messenger comparable to calcium
[65][66]; thus, it is obvious that cellular signals are altered due to changed intracellular zinc concentrations. Therefore, zinc is biologically indispensable for cellular processes, including growth and development, as well as DNA synthesis and RNA transcription
[64]. Additionally, zinc contributes to red-ox homeostasis because oxidative stress induces zinc release from metallothioneins as a mechanism to reduce ROS generated by mitochondrial dysfunction or viral infection
[67]. Furthermore, zinc deficiency increases IL-6-induced activation of the JAK-STAT3 signaling pathways, which are normalized after zinc supplementation
[68]. Zinc is known to be essential, especially for proper T cell and B cell development. During zinc deficiency, the recruitment of naïve Th cells and the percentage of cytotoxic T lymphocytes precursors is diminished, respectively
[69]. Zinc inhibits NF-κB signal transduction with the consequent decreased expression of IL-1β and TNFα and decreased CRP levels, lipid peroxidation, and inflammatory cytokines and adhesion molecule expression
[70]. More importantly, in the context of COVID-19 pathogenesis and its long-term consequences, zinc mediates the reduction of pro-inflammatory Th17 cells
[71], and its deficiency increases endothelial dysfunction
[72] and autoimmune susceptibility in general
[73]. It has been repeatedly demonstrated that autoimmune diseases are associated with zinc deficiency
[74], and an overreacting immune response can be beneficially influenced by the administration of zinc, which seems to be promising to improve the life of patients suffering from autoimmune diseases
[75]. In addition, several studies have documented a positive association between zinc deficiency and the risk of depression, and an inverse association between zinc supplementation and depressive symptoms
[76].
3.3. Phytochemicals: The Low Hanging Fruit
Viruses probably appeared as parasites of the first bacterial cells over 3.5 billion years ago
[77], while plants and homo sapiens appeared on earth 450 million and 300,000 years ago, respectively
[78]. Therefore, plants have hundreds of millions of years of greater experience in antiviral defenses than animals, and have most likely developed effective and non-specific defenses, i.e., valid against different viruses. Phytochemicals are naturally occurring plant chemicals that have been used in traditional medicines since ancient times and comprise various bioactive compounds that have now been classified as Alkaloids, Polyphenols, Carotenoids, and Organosulfurs, which are considered a natural weapon against inflammation and oxidation-mediated diseases
[79]. Natural substances contained in fruit and vegetables, such as resveratrol, quercetin, sulforaphane, and curcumin, to name but a few, all have a stimulating effect on the intranuclear pathway of transduction of the Nrf2 signal and an inhibitory effect on the NF-κB pathway (
Figure 1)
[80], with the result of limiting the effect of the cytokine storm that occurs in patients with severe COVID-19
[81] and persistent inflammation and autoimmunity
[82] that may occur in long COVID-19. These substances also exert an antiviral effect, both by binding to viruses outside the cell with electrostatic charges, and by preventing the binding process with their receptor, but also by limiting intracellular viral replication and hindering the escape of newly formed viruses from the cell
[45]. In fact, the addition of resveratrol to cell cultures infected with the SARS-2 virus prevents its replication and the consequent cell damage
[83] in relation to the conformational isomeric interaction between this polyphenol and the enzymes that all viruses use to replicate. The same inhibitory effect has been documented for quercetin
[84] sulforaphane and curcumin
[85]. The central structure of many synthetic antivirals consists of three rings present in most polyphenols
[86].