People are exposed to contaminants through the respiratory tract and skin; they first reach the bloodstream and, subsequently, the organs, causing more or less serious damage to health. Thus, the effects of atmospheric pollution affect the respiratory tract with acute symptoms and the circulatory system with cardiovascular events, leading to hospitalizations and mortality. In addition to the acute effects, long-term effects can also be had, including an alteration of lung function in adults, children, and adolescents. Specifically, in children and adolescents, chronic exposure to air pollution is associated with a reduction in forced vital capacity (FVC), which correlates with age and can be interpreted as a reduction in the lung growth and respiratory function of the lower airways. Children, together with elderly persons, are the most sensitive subjects to environmental pollution; to these are added subjects with chronic respiratory diseases such as asthma and chronic obstructive pulmonary disease (COPD). Emerging contaminants induce pulmonary toxicity by promoting an inflammatory response in lung epithelial cells.
1. Introduction
There are two main types of air pollution: pollution of the external environments (outdoor) and pollution of the domestic environments (indoor)
[1]. Today, air pollution is a current problem, since it can cause damage to the environment in which human beings live and to human health
[2]. Air is a mixture of fundamental components (78% nitrogen and 21% oxygen) and secondary components (1% carbon dioxide and other gases). Environmental pollution is due to the presence of elements in the air deriving from human activities (industrial sources, domestic heating, vehicular traffic) and from natural phenomena (e.g., volcanic eruption). These last natural sources can produce elements potentially harmful for the human respiratory system.
The substances that modify the composition of atmospheric air, causing pathological alterations for the respiratory system, are numerous and of different nature. In the recent decades, the number of subjects with chronic respiratory diseases increased exponentially in relation to air pollution
[3][4][5][6]. Already in the 1960s, when the main source of air pollution (outdoor) in cities was the combustion of coal, a close association between urban pollution and symptoms of bronchitis was observed, which was generally accompanied by decreased respiratory functions
[7]. An analysis from the
Global Burden of Diseases Study stated that air pollution is the second most common cause of death and disability for people with COPD
[8].
Air pollutants are largely produced by vehicle exhausts and are represented by carbon monoxide (CO), nitrogen oxides (NOx), sulfur dioxide (SO
2), polycyclic aromatic hydrocarbons (PAH), particulate matter (PM) and suspended powders, cadmium
[3], ozone, and volatile organic compounds (VOCs), which include benzene, toluene, and xylene, among others. Note that environmental air pollutants also include heavy metals such as lead, aluminum, and mercury
[4]. It is worth particular attention that carbon monoxide (CO), once inhaled, binds to hemoglobin, a protein of red blood cells responsible for transporting oxygen, forming carboxyhemoglobin (COHb). This bond is much more stable (about 200–300 times) than hemoglobin and oxygen; in this way, the CO prevents the normal transport of oxygen to the tissues, thus creating toxicological effects of different magnitudes
[9]. In fact, recent studies have reported that short-term exposure to environmental CO determines the increased risk of developing respiratory diseases (bronchiectasis, pneumonia, and asthma)
[10]. As for nitrogen oxides (NOx), they are compounds of both natural origin (volcanic eruptions and soil emissions) and anthropogenic, and their ability to form nitric acid in the mucosa of the airways and on the skin makes them toxic to humans and animals.
Short-term exposure to nitrogen dioxide (NO
2), especially in subjects with pre-existing lung disease, causes an increase in the exacerbation and an increase in bronchial reactivity. In addition, some meta-analysis studies have reported an association between NO
2 concentration and mortality from lung cancer
[11] as well as respiratory and cardiovascular diseases
[12]. Sulfur dioxide (SO
2) also causes harmful effects on human health. Exposure to this substance for a short period result in a significant increase in hospital admissions for respiratory diseases; 10 out of 25 are associated with an increase in symptoms and a reduction in lung function, while long-term exposure causes premature death and impairs the function of the airways, causing heart problems
[13][14].
Polycyclic Aromatic Hydrocarbons (PAHs) is a class of numerous organic compounds structurally characterized by the presence of two or more aromatic rings condensed together. PAHs include benzopyrene, acenaphthylene, anthracene, and fluoranthene, among others, and according to the Environmental Protection Agency, benzo-[a]-pyrene (B [a] P) is the most potent. PAHs are different for environmental sources and chemical characteristics. However, PAHs are formed during the incomplete combustion of organic products such as coal, oil, gases, or waste. They are present in the air as mixtures of many dozens of molecules, which are often present in different and variable proportions. The presence of PAH mixtures in the air makes it difficult to understand the specific mechanisms by which PAHs affect human health. These compounds are recognized as toxic, mutagenic, and carcinogenic and are an important risk factor for lung cancer
[15]. At the same time, the International Cancer Research Agency (IARC) identifies lead, cadmium, nickel, and arsenic as the most representative heavy metals for environmental risk and, both for their massive use and for their toxicity, are classified as carcinogenic to humans. Among these, nickel can have negative effects on the respiratory system, while lead is absorbed by the pulmonary epithelium and enters the bloodstream and is deposited in various organs
[16]. Therefore, the current legislation (Legislative Decree 155/2010) has defined a limit value for lead, arsenic, cadmium, and nickel in the air. On the other hand, fine powders (e.g., PM
10 and PM
2.5) are polluting particles present in the air, of organic or inorganic nature, capable of adsorbing on their surface various substances with toxic properties, such as sulfates, nitrates, metals, and volatile compounds.
The toxicity of these substances to the respiratory system increases as their size decreases. However, air contaminants are also present in the confined (indoor) environments where we live and work on a basis daily (formaldehyde, radon, volatile organic compounds, polycyclic hydrocarbons, etc.)
[3], and numerous studies have documented a link between increased mortality cardiovascular or respiratory and exposure to fine particulate matter (PM
10 and PM
2.5)
[17][18][19][20]. Furthermore, it has been shown that elevated concentrations of atmospheric PM are associated with an increase in hospital admissions for heart or respiratory diseases in subjects at risk (e.g., patients with asthma), and that chronic exposures to these substances are a risk factor for cancer
[21][22]. Respirable “particulate matter” (PM) has been identified by the US Environmental Protection Agenc as a “criteria air pollutant” together with carbon monoxide, ground level ozone, nitrogen dioxide, sulfur dioxide, and lead
[23][24][25].
In this regard, the European project ESCAPE (European study of cohorts for air pollution effects), which included nine European countries including Italy, highlighted the relationship between lung cancer, the degree of pollution in the areas of residence, and exposure to fine dust (PM
10 and PM
2.5). However, increases in lung cancer cases were also recorded in groups exposed to pollution levels below the maximum limits established under current European legislation (equal to 40 μg/m
3 of PM
10 and 25 μg/m
3 of PM
2.5)
[26]. Therefore, the World Health Organization (WHO) defined that it is not possible to establish a threshold value below which PM
2.5 is not harmful to people; therefore, the environmental concentration of PM
10 and PM
2.5 in the air should be kept as low as possible
[27]. PM
10, PM
2.5, and nitrogen oxides (NOx) are among the main atmospheric pollutants considered persistent carcinogens. They are monitored at European levels; about 90% of city dwellers are exposed to concentrations of pollutants higher than the values considered harmful to health
[28][29]. Furthermore, a recent study showed a close relationship between increased atmospheric concentrations of nitrogen dioxide and PM
2.5, death rate, and hospital admissions for COPD
[30], while lung cancer cases have been linked to the presence of PM in the deepest part of the lungs
[31]. The composition of PM mixtures from underground railways is very different of PM mixtures from urban areas. PM mixtures from underground railways are rich in metals associated with wheel, track, and brake wear and electrical arc and component wear
[32]. When particulate matter enters the respiratory tract, an interaction is created between lung epithelium and the immune system: this activates the local inflammatory response associated with the disease
[33]. Epidemiological and experimental studies suggest that among particulate air pollutants, diesel exhaust particles seriously affect the increase in morbidity and mortality from respiratory diseases. In fact, in urban areas, fine particulate matter produced by diesel engines (diesel exhaust particle cells) is a major source of PM
2.5 as it is readily inhaled deep into the lungs and remains there for a long time, resulting in cellular responses that generate intense inflammatory reactions in the airways
[32].
Respiratory diseases are multifactorial and therefore have various risk factors, including active and passive smoking, air pollution
[34], and cellular oxidative stress related to environmental contamination. This last is involved in the deregulation of cellular senescence and in severe airway disease with public health implications affecting the respiratory system
[35]. In the sites characterized by the presence of large industrial settlements, refineries of petrochemical nature, or chemical plants, there are the atmospheric pollutants. The air pollution can affect the respiratory system, causing malignant tumors or chronic inflammatory diseases of the lung in both adult and asthmatic children with a consequent increase in hospitalizations for asthma at a young age
[35].
Among the most dangerous compounds for human health, Persistent Organic Pollutants (POPs) should be mentioned, which are halogenated compounds persistent in the environment. This family of pollutants includes polychlorinated or polychlorinated biphenyls (PCBs), organochlorine pesticides, and polybrominated diphenyl ethers (PBDEs), which are all highly toxic. As for PBDEs, these are known as emerging contaminants generally referred to as “flame retardants”. According to the European Food Safety Authority (EFSA 2011), the main source of PBDE exposure is food of animal origin with high fat content (fish, meat, and dairy products)
[36]. They are ubiquitous and lipophilic, and they tend to accumulate in adipose tissue and interfere with the immune system
[4]. Therefore, humans are exposed to PBDEs through diet as well as through the accidental ingestion of dust, skin contact, and inhalation. Atmospheric levels of PBDEs depend on deposition processes, weather conditions, long-range atmospheric transport, and the proximity of PBDE sources to the sampling site (urban/industrial or background locations). A recent study monitored atmospheric PBDE levels (PBDE 28, 47, 85, 99, 100, 153, 154, 183, and 209) in Central Europe, and the results indicate a global increase in low-bromine PBDEs in atmosphere
[37]. This effect is due to the photolysis process, which favors the de-bromination of PBDEs with a higher bromine content.
Emerging contaminants such as the flame retardants (polybromo-diphenil, PBDEs ethers) PBDE-47, -99, and -209 are widespread in indoor and outdoor environmental contamination. These substances are present in fabrics, electrical materials, dust; e.g., they induce pulmonary toxicity by promoting an inflammatory response in lung epithelial cells
[38][39][40]. The same PBDE profile has been described in southern Europe by Besis et al.
[41], while Pozo et al. identified, of 26 PBDEs routinely analyzed, the presence of three (PBDE-47, -99 and -100) in the coastal areas of Sicily
[42]. These substances are highly carcinogenic, and they certainly interfere with the endocrine system (endocrine disruptors). They have a negative effect on the reproductive system and, in mouse study models, alter the immune system
[43], reducing the reactivity of macrophages compromising the associated immune response to cellular signals regulated by the TLR4/NF-kB pathway. A further experimental study showed that congeners -47, -99, and -209 are transferred from the mother to the fetus via the placenta, representing a risk factor for the development and growth of the fetus
[44], often leading to the development of child allergic/asthmatic phenotype
[4]. Due to their presence in the environment and their proven toxicity, commercial blends of Penta and Octa-PBDE have been banned in the European Union (EU) and some US states since 2004
[45] and in Canada since 2006. In 2009, they were designated as new persistent organic pollutants (POPs), and the Stockholm Convention banned their production and established the decrease in the use of commercial blends with more than seven bromine atoms (UNEP, 2015). Furthermore, in 2008, the EU banned the use of Deca-PBDE in electronic and electrical applications at concentrations higher than 0.1% of the total concentration of PBDEs
[46].
2. Cell Systems to Study the Effects of Environmental Contaminants in Respiratory Diseases
One of the “gold standards” of environmental scientific research is to obtain data to understand the effects of exposure to inhaled toxic substances on human health. In vivo
studies were used only to collect data related to an indirect effect of pollutants. These studied do not establish a direct relationship between ethical and safety precautions, high costs, very long periods, and environment with pathogenetic alterations regarding human health. Furthermore, data obtained from observational studies of subjects in the areas of environmental contamination are to lower the resolution of pathological effects at the cellular and molecular levels
[47].
For many years, scientific research has used animal models as the main tools to evaluate the effects of inhaled substances on human health. However, results obtained in mouse models are not always able to predict diseases, and their general use for research purposes has raised growing public and animal welfare concerns. The scientific research pushes toward the use of alternative and innovative in vitro/ex vivo experimental models
[48][49][50][51]. The history of experimental models began in 1885 with the zoologist Wilhelm Roux. He was the pioneer of experimental embryology studying the embryonic chicken cells in saline for several days. However, only in the mid-1950s, Harry Eagle gave a significant boost to this area of research by studying and identifying the nutrients needed by cells in culture
[52][53]. To date, the cell cultures and in vitro/ex vivo models are essential for the identification and the study of the effects of inhaled
noxae, which are represented by atmospheric pollutants, on biological systems
[54]. In recent decades, tissue engineering approaches have made enormous progress, and several in vitro models have been established to study the effect of inhalation toxicity and disease. The aims of these studies are to improve the understanding of pathophysiological processes and to provide new and more independent experimental systems for pharmacological and toxicological studies.
In vitro lung models are currently available for all important segments of the respiratory system starting from the nasal cavity and trachea to the proximal and distal airways
[55]. Traditional two-dimensional (2D) monolayer culture models (also called “submerged cultures”) are used to identify molecules involved in the signaling of altered cellular and molecular mechanisms that arise in the case of pulmonary toxicity. However, these models lack the key features of the human airway microenvironment that are essential for accurately studying the toxic effects of inhaled exogenous
noxae.
Recently, the traditional method of two-dimensional single-layer cell culture was replaced with more innovative methods, since it does not faithfully reflect what is observed within tissues in vivo
[56][57]. The main reason for the inadequacy of these cell culture systems is the lack of the architectural support and heterogeneity typical of the cells of the lung tissue. This awareness has led to an increase in the development of more complex three-dimensional (3D) models in which cells can grow in multiple directions in order to better reflect the cellular interaction present in the natural environment in vivo. Furthermore, multiple cell types may be present in these models
[39][58][59][60][61] and an extracellular matrix, thus allowing to mimic a model similar to one in vivo. The “
air–liquid interface” (ALI) culture is an example of these innovative models of cell culture (
Figure 1).

Figure 1. Three-dimensional (3D) ALI cultures of epithelial cells (primary and cell line) to study the effects of environmental contaminants in airway disease. Steps to obtain primary epithelial cells from human tissue: (a) Collection of bronchial biopsies or surgical specimens; (b) epithelial cell processing: tissue was dissociated, resuspended in bronchial epithelial growth medium; (c) cell expansion and culture of epithelial cells; (d) the cells are seeded onto the microporous membrane pre-coated with collagen in submerged conditions until confluence and culture in ALI; (e) reaching the confluence, the cells begin to lift at the air–liquid interface starting the differentiation fed by the culture medium in the basolateral side. Hence, the epithelial cells differentiate in the pseudostratified phenotype and build a tissue such as the epithelium of the lung.
This biological system of epithelial cells has a well-differentiated epithelium similar to human airways. Thus, epithelial cell cultured in ALI represents a valuable tool for scientific research to study the toxic effect of inhaled chemicals on the human health affecting respiratory diseases, providing the opportunity to evaluate and identify important cellular and molecular mechanisms
[62].
Airway epithelial cells cultured in ALI grow and form a pseudo-stratified epithelium composed of basal, ciliated, and goblet cells typical of a human airway epithelium in vivo
[63][64][65][66]. In this culture model, the cells fully differentiate, show tight junctions and cilia, and secrete mucins and protective mediators (e.g., antimicrobial peptides and pro-inflammatory cytokines), representing the in vivo structure and function of the airway epithelium
[64]. Thus, the ALI cultures have led to important advances in the characterization of cell biology, in the study of infections, in pharmacological and inhalation toxicity tests of the respiratory epithelium
[66][67]. ALI cultures mimic the human airway epithelium and successfully reproduce the lung microenvironment. Furthermore, these cell cultures can punctually reproduce the tissue of origin containing different structural (fibroblasts, endothelial cells, etc.) and inflammatory cell types (macrophages, neutrophils, eosinophils, etc.), and other than polarized epithelial tissue
[68]. Currently, there are various 3D organ-typic cell cultures (pulmonary micro-organ) of the airways that aid to understanding many cellular and molecular aspects of the effects of inhaled substances in the lung (
Figure 2)
[69].

Figure 2. Two different 3D organ-typical cell cultures to study the effects of environmental contaminants in airway diseases of the lung. (a) Three-dimensional (3D) cell culture model obtained with a co-culture of epithelial cells and fibroblasts; (b) Three-dimensional (3D) cell culture model obtained with multiple cell types from the pulmonary system (structural and inflammatory). Three-dimensional (3D) tetraculture system containing macrophages, epithelial cells, fibroblasts, and endothelial cells, mimicking lung organization.
Organ-typic cell cultures or organoids are defined as “cultured structures”. They are built with multiple cell types grouped and spatially organized in a similar way to the organ and show some organ functions
[70]. Organ-typic cell models may be obtained with commercially available materials of tissue culture. They are suitable for a variety of experimental projects and for modeling complex lung toxic responses, including inflammation, oxidative stress, myofibroblast formation, transepithelial migration, and invasion. Organ-typical patterns also mimic epithelial barrier properties and changes in cytotoxic and pro-inflammatory effects upon exposure to environmental toxicants such as those observed in vivo
[71][72][73].
The term ‘‘organoid’’ defines the 3D culture referring to stem cell-derived native-like tissue structures of a given organ created by the induction of genetically encoded self-assembly programming
[74]. Similar to processes that regulate organogenesis during embryonic development, cells within organoids undergo self-organization guided by cell-specific adhesion properties and spatially restricted progenitor differentiation. Organoid culture derived from stem cells or organ-specific progenitor cells that differentiate and self-organize through
cell sorting and
lineage commitment similar to the in vivo process. It should be noted that this recent definition is not yet strictly used in the literature
[75][76].
The gold standard of respiratory system modeling is represented by the lung-on-a-chip (LOC). It is the result of the combined use of most modern techniques of microfluidics and tissue engineering
[77][78]. The LOC model provides a microfluidic perfusion system to emulate the cellular microenvironment within the lung with high spatiotemporal precision
[77][78]. It is a device made on a transparent glass microplate and made up of three parallel microchannels. The central one is divided into two halves, lower and upper, by a porous membrane, on the two sides of which there are two layers of human cells, respectively endothelial cells of the capillaries and cells of the lining of the lung alveoli. Therefore, half of the channel functions as an airway and half functions as a blood vessel. The two channels are in close adhesion to each other, just as in an in vivo lung. Instead, the two lateral channels have a mechanical function, and when the vacuum is created in the device, they simulate a microdiaphragm
[79][80][81]. The LOC system offers new possibilities for inhalation toxicology research. These new technologies may represent an added value in the research; however, these tools are not yet widely available in the scientific community. In fact, technical problems in the use of induced pluripotent stem cells (IPSC) and LOC technology limit their use in the studies of inhalation toxicology
[81]. The successful applications of these innovative cell models in pulmonary drug discovery suggests their use in the assessments of inhalation toxicity. Therefore, future research aspires to the validation of innovative models and to the development, optimization, and implementation of new integrated cell models (
Figure 3).

Figure 3. Cell-based in vitro lung models for the study of inhalation toxicity. Visual representation of three-dimensional in vitro models of each model system for the study of inhalation toxicity: (a) organoid culture and (b) microfluidic and microfabricated device culture. ECM, extracellular matrix.
Many respiratory diseases begin with the onset of inflammatory reactions that play a key role in pathological lung conditions including asthma, COPD, and interstitial lung disease
[82][83]. The airway epithelium is the first line of defense against respiratory lesions from pathogens and toxic agents, as a functional barrier but also to initiate and amplify the immune response
[84]. Accordingly, herein have focused the attention on the effects of environmental pollutants on the epithelium of the respiratory tract, and below concluded mainly on some data relating to the simple cell models of 3D ALI cultures.
In recent decades, the exponential use of nanomaterials induces an increased risk of human exposure to nanoparticles (NPs)
[85]. Lenz et al. (2013) studied the effect of exposure to zinc oxide (ZnO) NPs present in the air in a double in vitro model of the submerged and ALI culture of human alveolar epithelial cells (A549 cell line)
[86]. It describes that the ALI culture of A549 exposed to ZnO-NPs showed a significant consistent cell response in terms of oxidative stress and inflammation compared to “submerged” cultures of A549. These data together with other references suggest that screening for NP toxicity in vitro with ALI models could produce better results than the results obtained with “submerged” cell cultures
[24][25][87][88]. Some studies show that chronic or long-term exposure to gaseous air pollutants may be responsible of long-term respiratory effects such as asthma, allergy, and even the onset of neurological disorders
[88]. Formaldehydes, carbon monoxide, and ozone are compounds commonly detected in indoor environments and are responsible for the development of acute toxicity (e.g., respiratory irritation)
[88][89]. However, the exposure of the cells to gaseous irritants is obtained with traditional “submerged” in vitro model
[90], and the compounds were added to the cell culture medium in liquid form. However, the chemicals added in the medium can alter their properties related to the interactions and binding of components of the medium. This might generate unreliable results
[91]. Recently, to test the effects of gaseous compounds on the cells of the airway, the use of an in vitro cell model of ALI cultures is recommended, as they are systems capable of mimicking gas exposure
[92]. To date, there are still few commercially available exposure systems that allow studying the effect of gaseous compounds in cell cultures with a precise dosimetry and without any interfering means
[93]. Ahmad et al. compare the chlorine toxicity using two epithelial cell models: the “submerged” models and the ALI models
[39]. It shows that chlorine reacts rapidly with aqueous surfaces to form hydrochloric and hypochlorous acid and demonstrates the toxicity of hydrochloric acid rather than chlorine in epithelial cells cultured in submerged conditions
[94]. In contrast, the exposure of human airway epithelial cells in differentiated ALI cultures allows a direct interaction between chlorine gas and cell surface in the absence of aqueous media. This type of cell culture is comparable to the realistic exposure scenarios put in place, following the inhalation of gases in environmental and occupational settings.
Albano et al. studied the effect of PBDE-47, -99, and -209 using a 3D “in vitro” model of human alveolar epithelial A549 cells (immortalized cell line) or human primary bronchial cells
[95] cultured in ALI. In which, the toxicity of PBDEs was studied with experimental procedures that mimic the exposure of the respiratory epithelium to inhaled contaminants. The data show that PBDE-47, -99, and -209 influence the physiological and biochemical mechanisms of oxidative stress (NOX-4 synthesis), cause inflammation (IL-8 synthesis, changes in the pH of cell fluids), and lead to the mucins’ overproduction and loss of pulmonary epithelial layer integrity measured by the transepithelial electrical resistance (TEER) and the expression of the tight junctions Zonula Occludens-1 (ZO-1). Furthermore, it provides encouraging evidence to describe a probable effect of the antioxidant N-acetylcysteine (NAC) on some pathological mechanisms generated by exposure to PBDEs in the airway’s epithelial cells. Therefore, the results support the concept that PBDEs could have negative effects on the respiratory epithelium physiology, promoting lung diseases in areas of environmental contamination. The model used represents an important platform for the screening of cell pathogenesis in human airways and turns out to be a powerful tool to improve knowledge of the effects of PBDEs on human health. Indeed, it highlights that the exposure of airway epithelial cells to PBDEs can generate oxidative stress, inflammation, pH acidification, mucus hypersecretion, increased viscoelasticity property, and loss of epithelial barrier function with subsequent alteration of its integrity (
Figure 4).
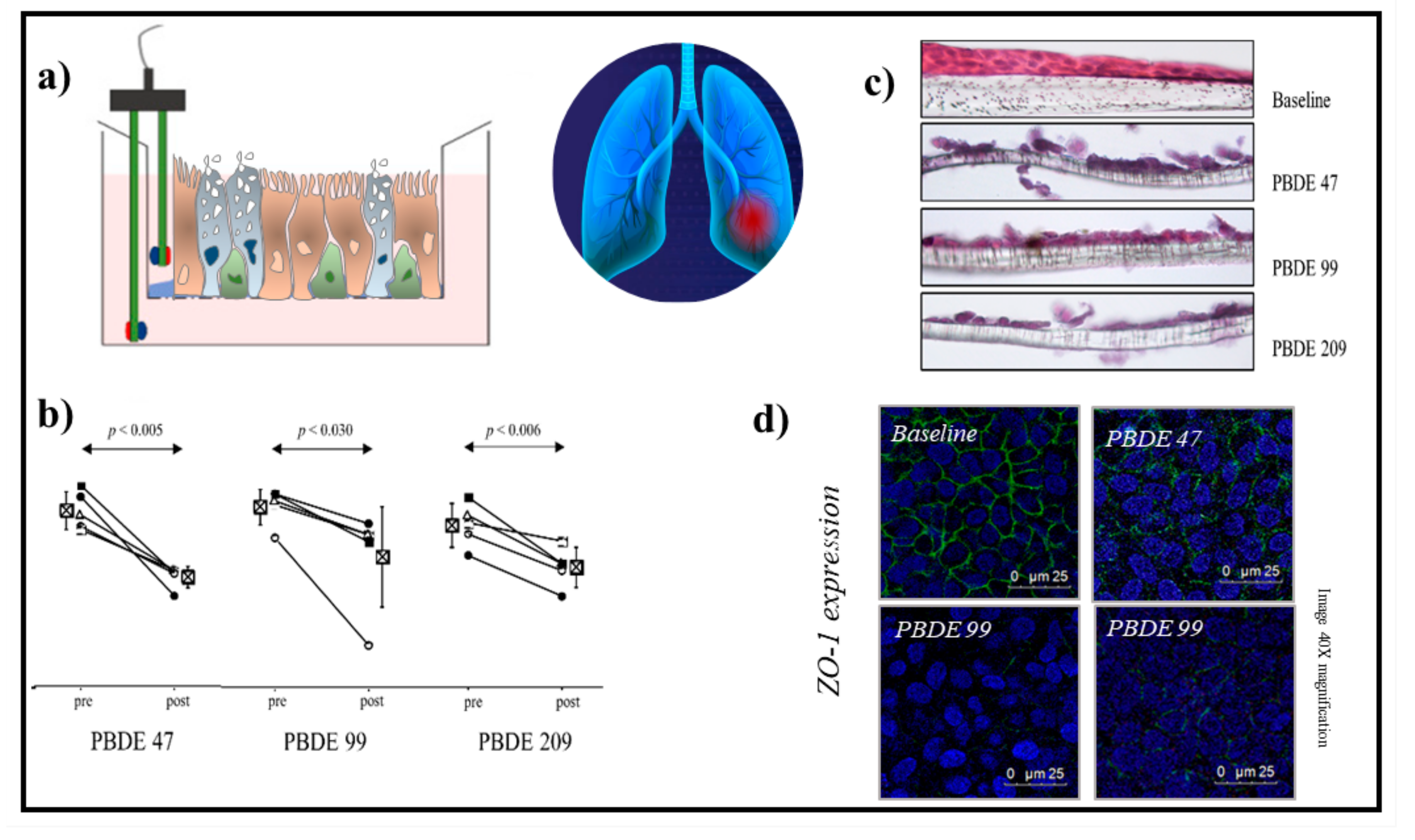
Figure 4. The measurement of TEER in the 3D ALI culture stimulated with air pollutants such as PBDE-47, -99, and -209 modifies the integrity of the epithelium of the lung, promoting airway diseases. (a) Electrode useful to measure the values of TEER in 3D cell culture; (b) reduction of TEER values before and after the treatment with contaminants of epithelial cells cultured in ALI; (c) morphological changes of epithelial cells cultured in ALI; (d) the damage of integrity of 3D epithelium is associated with a reduction of ZO-1 expression.
In fact, these markers of airway disease can be factors involved in the lung function decline, and their levels of the expression in the “in vitro” model of epithelial cells stimulated with PBDEs might suggest the increase of cases of acute and chronic lung disease in the areas of environmental PBDEs contamination (indoor, outdoor). About that, further in vivo studies would be needed to better elucidate the harmful effects of PBDEs on the pathophysiological complex of the airway epithelium as a cause of airway disease and related damage to human health. Therefore, to obtain results of better physiological relevance and understand the role of the airway microenvironment in response to chemicals inhalation, it is necessary to develop organ-typical (micro-organ) models capable of modeling 3D lung microenvironments using different type of lung cells organized in co- or multicultural systems.
3. Conclusions
Environmental contamination plays a fundamental role in human health, generating serious diseases such as inflammatory and neoplastic ones. Herein described some effects of air environmental contamination on human health, regarding lung diseases, and realized a descriptive approach with the aim to transfer the following in a simple way: (1) fundamental aspects of the activation of epithelial cells in respiratory diseases in case of exposure to contamination environmental; and (2) useful tools for an adequate in vitro/ex vivo experimental design to study the effect of air pollutants in the lung. About this last point, herein particularly refer to the experiences regarding the use of 3D ALI cultures of epithelial cells.
In this scenario, novel 3D in vitro models offer the advantage of enhanced physiological relevance through the incorporation of architectural support (i.e., ECM proteins or scaffolding), cell–cell interactions, and in some instances, biomimetic devices that can recapitulate physiologically breathing motions. However, despite their contributions, cell models have not been able to accurately represent the heterogeneity of the human population and account for interindividual variability in response to inhaled toxicants and susceptibility to the adverse health effects.
Herein focalized the attention to the concerns about the effects of air environmental contamination on human health regarding diseases of the lung with particular attention to the role of epithelial cells. The descriptive approach has been discreet with the aim to transfer in a simple way the fundamental aspects of the activation of epithelial cells in respiratory diseases in case of exposure to environmental contamination. Here refer to the experiences about the use of 3D ALI cultures of epithelial cells to study the effect of some toxicants on epithelial cells.
Furthermore, here introduced the concept of the exposome, since it constitutes a new paradigm for studying the impact of the environment on human health. Finally, the contribution of Omics Sciences defined new scientific perspectives aimed at the discovery of the cellular and molecular mechanisms underlying the immunological response of the airway epithelium in conditions of environmental air contamination. The goal of the researchers might be to enrich the concept of exposome using innovative biological systems that mimic organ situations in real life.
In conclusion, with the short descriptions enclosed here, it is underlined and suggested the importance of planning new technologic and conceptual perspectives useful to further clarify the effect of environmental factors on the health of the lung. The specific objectives to be achieved are to identify new cellular and molecular pathways associated with the concept of the exposome, with the help of complex organotypic and organoid cultures with applications of microfluidics and omics sciences.