Transient Receptor Potential (TRP) channels constitute a large superfamily of polymodal channel proteins with diverse roles in many physiological and sensory systems that function both as ionotropic and metabotropic receptors. From the early days of TRP channel discovery, membrane lipids were suggested to play a fundamental role in channel activation and regulation. A prominent example is the Drosophila TRP and TRP-like (TRPL) channels, which are predominantly expressed in the visual system of Drosophila. Light activation of the TRP and TRPL channels, the founding members of the TRP channel superfamily, requires activation of phospholipase Cβ (PLC), which hydrolyzes phosphatidylinositol 4,5-bisphosphate (PIP2) into Diacylglycerol (DAG) and Inositol 1, 4,5-trisphosphate (IP3).
1. Introduction
The Transient Receptor Potential (TRP) superfamily, which is conserved through evolution, consists of seven subfamilies (TRPC, TRPV, TRPM, TRPA, TRPN, TRPML, and TRPP) and its members are expressed in many cell types, including excitable as well as non-excitable cells
[1]. These channels participate in many sensory modalities (e.g., vision, taste, temperature, pain, and pheromone detection) and they either open directly in response to ligands or physical stimuli (e.g., temperature, osmotic pressure) or open indirectly, downstream of receptor activation (e.g., Rhodopsin, Histamine, and Bradykinin
[2][3]) and the inositol-lipid signaling cascade. In addition to the wide variety of activation mechanisms, the activity of TRP channels is modulated by numerous factors, including the lipid environment in which the channel is embedded, posttranslational modifications such as phosphorylation, nitrosylation and glycosylation, ligand biding such as Ca
2+ and ATP and by interaction of the channels with different binding partners
[4]. Despite many efforts over the years, the gating mechanism of the founding members of the TRP channel superfamily, the
Drosophila TRP and TRP-like (TRPL) channels is still elusive.
Visual systems of animals are characterized by high sensitivity to light and this property is obtained by a high expression level of the signaling components within the retinal cells. The retina of
Drosophila is an excellent example of this principle as revealed by expression of huge amounts of signaling components and by achieving the ultimate sensitivity to light–single photon detection.
Drosophila utilizes the phosphoinositide cascade for phototransduction with TRP channels as its target
[2][3]. Hence, the
Drosophila retina is a unique tissue with regard to TRP channels, as most tissues do not express high amounts of TRP channels. This TRP-enriched tissue enables robust biochemical analysis combined with the power of the
Drosophila molecular genetics and the accuracy of light activation and constitutes a valuable preparation for investigating the complex mechanism of TRP channel gating under physiological conditions. Despite having many advantages, the
Drosophila photoreceptor preparation still has several limitations, including the polarity of the
Drosophila photoreceptor cells and the dense membrane of the signaling compartment (rhabdomere), which limits pipette accessibility to the channels, making it difficult to directly determine the single channel properties under physiological conditions
[5].
Lipid regulation of channels is mostly thought as ligand-protein interaction with a defined binding site at the channel surface. However, recent in-silico lipid docking analysis using solved channel structures suggests that some lipid binding sites enable binding of multiple lipid “poses”
[6]. Another interesting theory developed for the gating mechanism of
Drosophila TRP/TRPL channels proposed that the TRP/TRPL channels are mechanically gated. Accordingly, the measured force generated by changes in membrane lipid packing and the generation of membrane tension during the enzymatic activity of phospholipase Cβ (PLC) and the conversion of PIP
2 into DAG, gate the mechanical sensitive TRP/TRPL channels. This theory emphasizes that the lipid environment in which the channels are embedded is crucial for their performance.
2. The Involvement of the Inositol-Lipid Signaling in TRP Channel Activation
One of the most important contributions of the
Drosophila retinal preparation to the study of TRP channels in general and of the TRPC channels subfamily in particular is the discovery that Gq-mediated phospholipase Cβ (PLC) has an essential role in physiological activation of the TRP channels (
Figure 1). Evidence for possible participation of PLC in
Drosophila phototransduction arose from initial biochemical studies, showing that highly reduced PLC enzymatic activity was found in the no receptor potential A (
norpA) mutants
[7][8][9]. Further detailed functional evidence for a light-dependent G
qα-mediated PLC activity in fly photoreceptors came from combined biochemical and electrophysiological experiments. These experiments revealed coupling of photoexcited rhodopsin to phosphatidylinositol 4,5-bisphosphate (PIP
2) hydrolysis
[10].
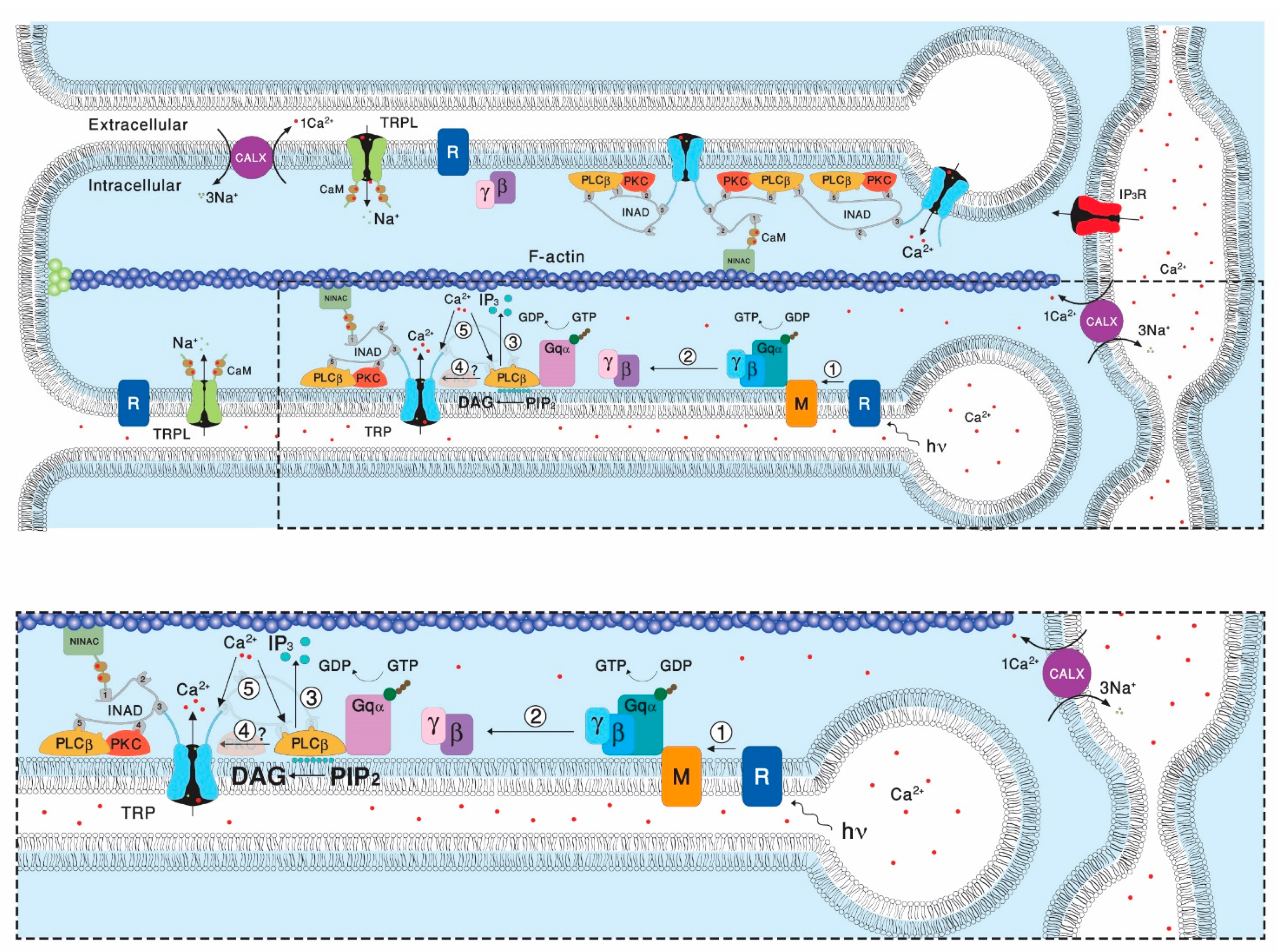
A reduction in the levels of PLC in mutant flies affect the amplitude and activation kinetics of the light response
[11], but surprisingly also induce the slow response termination. This slow response termination of most
norpA mutant alleles had no explanation for many years. Later biochemical and physiological studies conducted in
Drosophila revealed the requirement for PLC in GTPase-activating proteins (GAP) activity in vivo
[12], suggesting that the PLC enzyme also acts as a GAP
[13].
3. Lipid Composition of the Drosophila Head/Retina and the Effects of Its Modification
There is mounting evidence that lipids have profound effects on the activity of ion channels in general and on members of the TRPC subfamily in particular
[14]. Lipids of
Drosophila head membranes have been analyzed using thin layer chromatography, gas liquid chromatography
[15][16], and nano-electrospray ionization tandem mass spectrometry (ESI-MS/MS)
[17]. These studies revealed the presence of ~50% phosphatidyl-ethanolamine (PE), ~25% phosphatidyl-choline (PC), and ~12% phosphatidyl-inositol (PI). Contrary to vertebrate photoreceptors, long chain polyunsaturated fatty acids (more than 18 carbons) were not detected in
Drosophila phospholipids
[16]. In addition to phospholipids,
Drosophila head membranes contain sphingolipids and, instead of cholesterol, ergosterol
[17][18]. The ergosterol content of
Drosophila head membranes was determined by ESI-MS/MS and revealed ~26 nmol ergosterol per mg of membrane proteins
[17]. The sphingolipids are composed of a tetradeca-4-sphingenine and a saturated fatty acid
[18]. Since these studies used membranes of entire
Drosophila heads, the obtained quantitative results may not mirror the exact lipid composition of photoreceptor membranes.
Lipidomic analysis of fly heads raised on a yeast-free diet showed that the lack of Poly Unsaturated Fatty Acids (PUFAs) in this diet was reflected in marked differences in fatty acids composition of all the major phospholipids in the heads. In particular, while mono-saturated lipid species were little affected, polyunsaturated (with 3 or more double bonds) were greatly reduced in all classes of phospholipids.
4. Evidence for Lipids Action as Second Messengers
Many mammalian TRP channels can be characterized as ionotropic receptors since they can be activated directly under physiological conditions by physical stimuli such as heat, cold, mechanical, or by natural chemicals such as capsaicin, menthol, or mustard oil
[19]. One of the key features of presumably all members of the TRPC subfamily is their indirect physiological activation via PLCβ-mediated signal transduction cascades. Since PLC activity is essential for activation of the TRP/TRPL channels, recycling its substrate, PIP
2, is essential for proper light response. PLC catalyzes hydrolysis of the membrane phospholipid PIP
2 into water soluble IP
3 and membrane-bound diacylglycerol (DAG,
[20]). This enzymatic reaction is enhanced by illumination and initiates a cyclic enzymatic pathway called the PI cycle. Many enzymatic components of the complex PI cycle were discovered using genetic dissection by screening for mutations and utilizing the power of the
Drosophila molecular genetics
[21]. Following PLC activation, the phospholipid branch of the PI cycle begins by DAG transport through endocytosis to the endoplasmic reticulum designated submicrovillar cisternae (SMC). Subsequently, DAG is inactivated by phosphorylation and converted into phosphatidic acid (PA,
[22][23] via DAG kinase (DGK), encoded by the retinal degeneration A (
rdgA) gene that was discovered as a mutant causing rapid retinal degeneration in the dark
[22][23].
5. PUFAs Activation of TRP and TRPL Channels in the Dark
One of the striking findings of Hardie and colleagues in studies of
Drosophila photoreceptors was the demonstration that PUFAs such as linolenic or linoleic acid (LA) are potent activators of the TRP/TRPL channels in the dark. This robust, but still slower activation relative to light activation was demonstrated in both photoreceptor cells
[24][25] and in cultured S2 cells (
[24][25]) and HEK cells
[26] heterologously expressing TRPL channels.
A question arises as to whether PUFA activation of the channels in the photoreceptor cells reflects the physiological mechanism of channel activation. Supporting evidence was provided by isolation of the Inactivation No Afterpotential E (
inaE) mutant by Pak and colleagues
[27]. The
inaE gene was identified as encoding a homologue of the mammalian
sn-1 type DAG lipase, which, rather than PUFAs, releases mono-acyl glycerol (MAGs) and, are at best, weak and slowly acting channel agonists when applied exogenously (Hardie, unpublished results, see
[28]). Furthermore, the
inaE gene product immunolocalizes to the cell body with occasional puncta in the rhabdomere
[27]. Mutant flies, expressing low levels of the
inaE gene product, have an abnormal light response, while the activation of the light sensitive channels was not prevented
[27]. The discovery of the
InaE gene was an important step in the endeavor to elucidate lipids regulation of the channels
[21]. However, for PUFA generation, either an sn-2 DAG lipase or an additional enzyme (MAG lipase) would be required, but there is no evidence of either in
Drosophila photoreceptors and there is also no evidence that PUFAs are even generated in response to illumination
[29].
6. Photomechanical Gating of the TRP/TRPL Channels
Many studies have shown that membrane-protein function is regulated by the composition of the lipid bilayer in which the proteins are embedded. The commonality of the changes in protein function by changes in their lipid environment suggests an underlying physical mechanism, and at least some of the changes are caused by altered bilayer physical properties
[30]. Although TRP/TRPL channel activation by PUFA is most likely not a physiological mechanism, it provides an important insight into mechanisms by which membrane lipid modulation by a variety of PUFAs causes dramatic effects on TRP/TRPL channel gating and its properties. Accordingly, it was previously showed that removal of open channel block (OCB) from TRP/TRPL channels by PUFA resulted from an increased flow rate of the blocking divalent cations through the channel pore
[25]. Modulation of the interface of the channel and its surrounding membrane lipids might underlie the increase in the flow rate of the blocking cations and the removal of the OCB. Various methods were applied to modify membrane lipid properties around the TRPL channel, including: stretch of the plasma membrane by hypoosmotic solutions, sequestration of PIP
2 by polylysine, and application of various lipids. Alternatively, PUFA action was blocked by the GsMTx-4 tarantula toxin, a specific inhibitor of mechanosensitive channels, which acts on the channel-membrane lipid interface
[31][32]. These results thus suggested that lipids do not affect the TRPL channel as second messengers but rather as modifiers of membrane lipid-channel interactions
[25].
7. Lipid Rafts and Modulation of TRPL Channel Activity by Cholesterol
Lipid rafts are membrane microdomains rich with sterols, sphingolipids, and specific proteins. Lipid rafts have been found in all types of cells. It has been generally agreed that lipid rafts generate signaling platforms by assembling in close proximity to different signaling molecules for the optimal function of signal transduction cascades
[33]. Many signaling proteins including ion channels have been found to localize in lipid rafts, while the association with rafts was required for the regulation of some integral membrane protein activities
[34][35]. The actions of lipid rafts are often associated with the effect of cholesterol on membrane structure or directly on membrane proteins. Channels have been found in detergent-resistant membrane (DRM) fractions, indicating the inclusion of channels in lipid rafts
[36].
8. Conclusions
Despite many efforts over the years, the gating mechanism of the Drosophila TRP/TRPL channels still poses a long-standing enigma. The findings that mammalian TRPC channels can be activated by exogeneous application of DAG together with the solved atomic structures of mammalian TRPC channels by cryo-EM constitute major progress towards understanding TRPC channel gating. Nevertheless, Drosophila retina is a highly valued preparation for investigating the roles of TRP/TRPL channels under physiological conditions. This is due to their high expression levels and their known functional role as the light-activated channels. There remains no comparable preparation for the mammalian TRPC channels that contains a TRP-enriched tissue combined with the power of the Drosophila molecular genetics and the accuracy of light activation. Moreover, studies of Drosophila phototransduction revealed that under physiological conditions, Drosophila TRP operates as an essential part of a multimolecular signaling complex. There are indications that mammalian TRPC channels also operate in a similar manner, but it is difficult to study these mechanisms in the native mammalian systems because of the scarcity of these signaling proteins in the native systems.
Crucial unsolved questions in
Drosophila photoreceptor physiology are related to the mechanism by which DAG accumulation following DGK inhibition activates the channels. In particular, why exogeneous application of DAG to an excised signaling membrane activates the channels in orders of magnitude slower than light activation in the intact photoreceptor cell. The use of photoactivated DAG analogues
[37] may help in solving this question.
An available Drosophila mutant in which the TRP channels are constitutively active (i.e., the trpP365 mutant) is a highly valuable tool for investigating TRP gating in the future, which can be used together with the solved atomic structure of mammalian TRPCs. In addition, even if PUFA is not the native second messenger of excitation, its robust effect of opening the TRP/TRPL channels in the dark in vivo and TRPL in tissue culture cells can be studied in detail and provide useful information on channel gating.
Finally, the photomechanical gating mechanism of TRP/TRPL channels that was put forward by Hardie and colleagues needs to be revisited. According to this model and the supporting evidence, light-induced PIP2 hydrolysis by PLC converts a phospholipid with a large hydrophilic head group (PIP2) into DAG with a minute hydrophilic head group. This enzymatic reaction leads to a generation of a measurable force that may open the channels mechanically.