The developments within the topic of biomaterials has taken hold of researchers due to the mounting concern of current environmental pollution as well as scarcity resources. Amongst all compatible biomaterials, polycaprolactone (PCL) is deemed to be a great potential biomaterial, especially to the tissue engineering sector, due to its advantages, including its biocompatibility and low bioactivity exhibition. The commercialization of PCL is deemed as infant technology despite of all its advantages. This contributed to the disadvantages of PCL, including expensive, toxic, and complex.
1. Introduction
Among several types of biopolymers, polycaprolactone (PCL) has received a lot of attention due to its several advantages, such as its biodegradability, high strength, and biocompatibility. It can withstand water, oil, solvents, and chlorine. PCL is a semicrystalline ester polymer that is derived from ring-opening polymerization of ε-caprolactone monomers, as shown in Figure 1. PCL consists of a glass transition temperature (Tg) of around 60 °C and a melting point ranging between 59–64 °C, dictated by the crystalline nature of PCL which enables easy formability at relatively low temperatures [1].
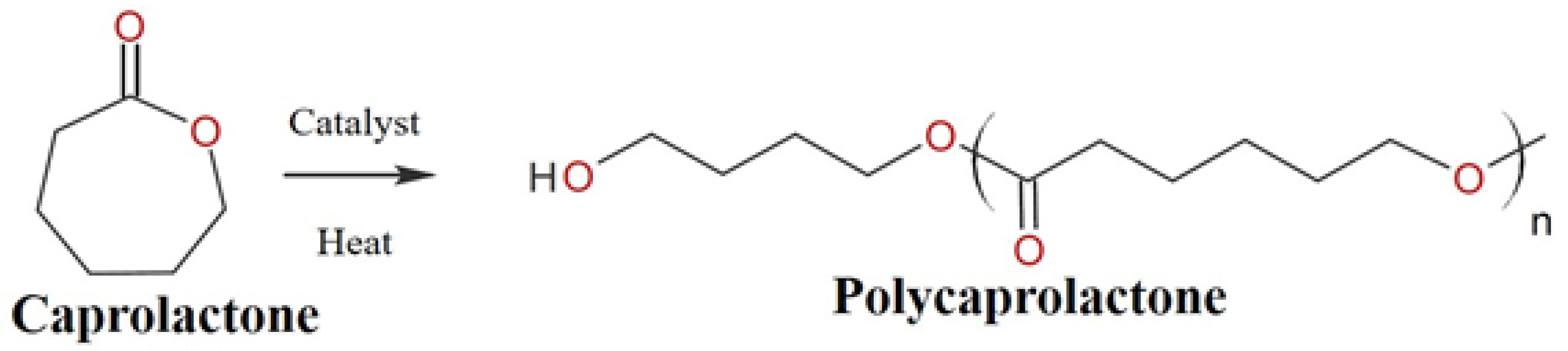
Figure 1. Synthesis of PCL.
In contrast, the average molecular weight of PCL samples can range from 3000 to 90,000 g/mol, and they can be classified based on their molecular weight. With increasing molecular weight, its crystallinity decreases. High solubility, a low melting point, and exceptional blend compatibility have sparked a lot of interest in its potential biomedical applications
[2].
Table 1 summarizes the effect of PCL molecular weight on their properties
[3].
Table 1. Properties of PCL with different molecular weights.
Molecular Weight |
Melting Point, °C |
Tensile Stress, N/m2 |
Elongation at Break, % |
37,000 |
58–60 |
1.37 × 107 |
660 |
50,000 |
58–60 |
3.53 × 107 |
800 |
80,000 |
60–62 |
5.69 × 107 |
900 |
PCL also shows great electrospinning properties, as it can be spun into fibers at temperatures around 200 °C, without undergoing thermal degradation. Since PCL is a synthetic material, it is possible to achieve high material purity. PCL also has relatively long biodegradable time. According to the literature, PCL can biodegrade in a few months to many years, depending on its molecular weight, degree of crystallinity, shape, porosity, sample thickness, and the surrounding environment
[4], unlike traditional plastics, such as polypropylene (PP) and polyethylene (PE), which take hundreds or even thousands of years to fully decay.
Recently, due to the environmental concerns, as well as energy and cost considerations, increased research efforts have been directed to the creation of bio-based materials. Bio-based polymers, which minimize reliance on petrochemical-based synthetic polymers, contribute significantly to global environmental sustainability. Recently, Gokhan et al. (2020)
[5] has synthesized bio-based PCL from soybean oil-derived polyol via ring-opening polymerization. He found that the soybean-based PCL containing higher PCL molar ratio has poorer biodegradability but higher hydrophobicity and thermal characteristics compared to the others. Nonetheless, biobased polymers possess several weaknesses, including inferior mechanical properties, insufficient heat tolerance, and high moisture sensitivity relative to petroleum-derived polymers
[6].
As mentioned in a previous statement, this polymer is well known for being biodegradable and have notably lower degradation. Due to its slow degradation rate, PCL is often used as a material in drug delivery devices that is active for a long time, with a period of over one year
[7]. The material is also noted to be potentially used as a bioscaffold
[8][9]. In terms of its biodegradability, permeability, and inability to establish an acidic environment, PCL is a better polymer than PLA or PGA in terms of possible biomedical uses
[10]. Furthermore, because of the low rate of PCL degradation compared to PLA and PGA, it can be utilized to release medications over longer periods of time, even a year. The hydrolytic cleavage of ester groups is involved in PCL degradation. It is easy to predict that inserting different co-monomer units in the PCL chain will result in diverse properties, including changes in biodegradability, allowing for biodegradability control through targeted introduction of such groups
[11]. Manivasagam et al. (2019)
[12] have mentioned the application of PCL in dentistry by modifying the material structure with other materials (such as hydrophilic polyethylene glycol (PEG) and ceramic) and introducing co-polymers (such as polylactic acid (PLA) or polyglycolic acid (PGA)). The pKa of the degradation products; the primary mechanisms of the degradation; and the in vivo degradation rate of PCL, PLA, and PGA are summarized in
Table 2.
Table 2. The degradation behaviour of the biodegradable polyesters. Reproduce from ref.
[13].
Polyester |
Degradation By-Products (pKa) |
In Vivo Degradation Rate |
Degradation Mechanism |
PCL |
Caproic acid (4.88) |
50% in 4 years 1% in 6 months |
Hydrolytic degradation |
PLA |
Lactic acid (3.85) Lactic acid (3.08) |
50% in 1–2 years 98% in 12 months 100% in >12 months 100% in 12–16 month |
Hydrolysis through the action of enzymes |
PGA |
Glycolic acid (3.83) |
100% in 2–3 month 100% in 6–12 months |
Both enzymatic and non-enzymatic hydrolysis |
Unfortunately, because PCL manufacture is both complex and expensive, its wider commercialization has been limited. In addition, the material adheres poorly to cells due to its hydrophobic surface. PCL solvents are also known to be toxic, which can potentially harm human beings. The relatively low melting point is also demonstrates another drawback, since it hinders the material from being applied at higher temperatures
[14][15][16].
Table 3 shows the summary of advantages and disadvantages of PCL.
Table 3. Advantages and disadvantages of PCL.
Advantages |
Disadvantages |
High biocompatibility |
Adheres poorly to cells |
Highly biodegradable |
Toxic solvent |
Great electrospinning properties |
Low melting point |
Long biodegradable time |
Complex and expensive production |
High material purity |
|
Nonetheless, those limitations of PCL can potentially be overcome by the use of PCL-based biocomposites. Extensive efforts have been made over the last decade in the development of PCL-based biocomposites. The properties of PCL can be improved by blending with other polymers or fibres, allowing more people to benefit from its excellent properties
[17][18][19][20]. Mechanical and thermal properties are the most influential, affected by the addition of fillers to the PCL
[21][22][23]. Several studies have shown that the PCL-based biocomposites can be benefited for several applications, especially in the biomedical field
[15][24][25][26][27][28].
2. Applications of Polycaprolactone-Based Biocomposites
PCL has been widely applied in biomedical fields, especially in tissue engineering and medical implants, because they are biodegradable and have high biocompatibility. Manivasagam et al. (2019)
[12] mentioned that polycaprolactones are being explored extensively in bone tissue engineering due to a lack of bioactivity and high degradation rates. Miller et al. (2011)
[9] have stated that PCL can potentially be used as a bioscaffold. Nevertheless, when it comes to the application of tissue engineering, PCL suffers from some shortcomings, such as poor mechanical properties, slow degradation rate, and low cell adhesion. The reinforcement of bioactive glasses and calcium phosphate-based ceramics within PCL has created a class of hybrid biomaterials with improved controllable degradation rates, good mechanical properties, and enhanced bioactivity, making them suitable for bone tissue engineering
[29]. PCL green biocomposites with superior mechanical properties can potentially be used as orthopaedic implants, as briefly outlined as.
The Food and Medication Administration (FDA) has approved PCL for use in specified applications in the human body as a suture, drug delivery device, or adhesion barrier
[7][30][31][32]. Following the recent launch of a PCL-based microsphere dermal filler belonging to the collagen stimulator class (Ellansé), PCL is being employed in the field of human aesthetics
[33][34]. PCL-based products have also been utilized to treat facial ageing indications, such as contour laxity and volume loss, by stimulating collagen formation, resulting in an immediate and long-lasting natural impact
[30][34][35][36][37][38]. In addition, it is being studied as a scaffold for tissue engineering-mediated injury repair using the guided bone regeneration (GBR) membrane, which has been extensively reported by numerous researchers
[39][40][41][42][43][44][45]. It has been utilized as a hydrophobic block in amphiphilic synthetic block copolymers that are used to build the vesicle membrane of polymersomes
[46][47].
Recently, PCL green biocomposites have been developed and commercialized. The majority of PCL green biocomposites are currently in the research and development stage. To make green biocomposites at a cheaper cost, new processing techniques and technologies are being developed
[48]. Hao and co-workers found that the twin screw extrusion was used to make PCL–CNC nanocomposites. Microcellular nanocomposite samples were created utilising microcellular injection moulding and a physical blowing agent of carbon dioxide (CO
2). The biocompatibility of the material was examined. The green hue in the florescence photographs symbolises live cells, whereas the red colour depicts dead cells. In comparison to bare dead cells, the clean PCL sample exhibits a substantial number of living cells. It was also found that 0.5% CNC and 1% CNC samples had mostly live cells, thus indicating good compatibility between the substrate and the cells.
PCL beads have been used to encapsulate a range of medicines for controlled re-release and targeted drug delivery
[49][50][51][52][53][54][55][56][57][58][59]. In addition to polymeric properties several other factors, such as the type and objective of formulation, the route of administration, drug or polymeric properties etc., also affect the selection of the polymer (
Figure 2). Hence, selection of a polymer is an important step for the development of a successful drug delivery system or device
[60]. PCL-based modified polymers demonstrated so far predominantly involve its copolymers with several other polymers in different forms. Nevertheless, other modifications (blends and composite) were also reported in different formulations
[61][62].
Figure 2. Factors affecting polymer selection.
Among biodegradable polymers modified for amelioration of properties, a special focus is made on PCL mainly due to its broad spectrum of compatibility with a wide range of other polymers. Its versatile nature, ease of fabrication, and biocompatibility establish it to be the polymer of interest by investigators worldwide for drug delivery and tissue engineering applications
[63][64][65][66][67]. However, when scientists consider properties of unmodified PCL, there are considerable restrictions for its use. For example, its hydrophobic nature does not allow the facile release of hydrophobic drugs (from prepared formulations) and micelle formation. Furthermore, long-term degradation (ranging from weeks to months) slows down tissue replacement in the case of scaffolds, mechanical property limits its application to hard tissue engineering only, and nonreactivity is unsuitable for preparation of NC. Therefore, attempts have been made to overcome these undesirable properties by various types of modification, for successful application in pharmaceutical formulations. To great excitement, the use of modified PCL dominated over the past decade by virtue of which PCL was demonstrated in almost all novel formulations overcoming the above-mentioned restrictions. Additionally, functionalization as a result of PCL modifications is a featured advantage and is considered as another cause for this typical preference for modified PCL.
Additionally, the possibility of using PCL to be used as implants for targeted drug delivery has been explored. Boia et al. (2019)
[68] have developed a novel way of using porous polycaprolactone as an intraocular implant to deliver dexamethasone to replace eye drops or intravitreal injections. The implants were made by using green supercritical carbon dioxide foaming or mixing methods, resulting in the implant having high porosity and high surface area. This, in turn, will cause a higher degradation rate than typical PCL-based implants, which have a relatively slower degradation rate. They are then inserted into adult rats for further observation. The implant is shown to have good biocompatibility, since it does not cause cell death or reduce the number of neurons
[68]. In contrast, Hivechi et al. (2019)
[69] investigated the regulated release of tetracycline hydrochloride using CNC-reinforced PCL nanofibers. The amount of CNC in the PCL nanofibers was increased, which resulted in a delayed release of a medication.
3. Conclusions
PCL is truly a promising materiel for the future to replace the current materials that are impossible and expensive to reuse. The aim is to investigate the potential of PCL biocomposites reinforced with natural fibres to enhance the quality of the produced biocomposites. Blending and processing of PCL into biocomposites has improved the materials’ properties, thus providing more area to exploit these excellent properties. Herein, green biocomposites and hybrid biocomposites of PCL were evaluated in terms of their mechanical and thermal properties. The characteristics and properties of natural fibres can greatly influence the final properties of PCL-based biocomposites. Most of the studies reported that the performance of the PCL biocomposites improved, as compared to the neat PCL after blended with natural fibres. Moreover, emerging uses of nanofillers, such as nanocellulose and MMT, also managed to greatly improve the performance of PCL-based green and hybrid biocomposites. Besides that, PC-based biocomposites have huge utilization and potential within drug delivery devices, medical devices, and tissue engineering.