Multiple myeloma (MM) is an incurable hematologic malignancy characterized by the clonal expansion of malignant plasma cells within the bone marrow. Activator Protein-1 (AP-1) transcription factors (TFs), comprised of the JUN, FOS, ATF and MAF multigene families, are implicated in a plethora of physiologic processes and tumorigenesis including plasma cell differentiation and MM pathogenesis. Depending on the genetic background, the tumor stage, and cues of the tumor microenvironment, specific dimeric AP-1 complexes are formed. For example, AP-1 complexes containing Fra-1, Fra-2 and B-ATF play central roles in the transcriptional control of B cell development and plasma cell differentiation, while dysregulation of AP-1 family members c-Maf, c-Jun, and JunB is associated with MM cell proliferation, survival, drug resistance, bone marrow angiogenesis, and bone disease. The present review article summarizes our up-to-date knowledge on the role of AP-1 family members in plasma cell differentiation and MM pathophysiology. Moreover, it discusses novel, rationally derived approaches to therapeutically target AP-1 TFs, including protein-protein and protein-DNA binding inhibitors, epigenetic modifiers and natural products.
1. Introduction
First described in the 1980′s [1][2][3][4][5], members of the Activator Protein-1 (AP-1) transcription factor (TF) family contain the characteristic basic leucine zipper (bZIP) domain, which enables dimer formation via a stretch of hydrophobic leucines, and facilitates DNA interaction via positively charged amino acids. AP-1 family members include the JUN (c-Jun, JunB and JunD), FOS (c-Fos, FosB, Fra-1 and Fra-2), ATF (ATF2, ATF3/LRF1, ATF4, ATF5, ATF6B, ATF7, B-ATF, B-ATF2, B-ATF3, JDP1 and JDP2) and MAF (MafA, MafB, c-Maf, Nrl and MafF/G/K) multigene subfamilies [6]. While Jun proteins heterodimerize or homodimerize with members of their own subfamily, Fos proteins must heterodimerize. Depending on their composition, AP-1 TFs bind to the TPA-response element (TRE) [5′-TGA (C/G) TCA-3′] and, with lower affinity, to the cAMP response element (CRE) [5′-TGA CG TCA-3′], which is almost identical to TRE. Specifically, Jun: Jun dimers and Jun: Fos dimers preferentially bind to TRE and CRE, but also to variant sequences within the DNA; ATF-containing Jun: ATF and ATF: ATF dimers preferentially bind CRE; and MAF-containing dimers bind either to MAF-recognition element (MARE) I [5′-TGC TGA (C/G) TCA GCA-3′] or to MARE II [5′-TGC TGA CG TCA GCA-3′], extensions of TRE and CRE sequences [1]. In addition, AP-1 dimers interact with non-bZIP proteins including CBP/p300, p65/NFκB and Rb. AP-1 activity is induced by a multitude of intrinsic and extrinsic stimuli and environmental insults including cytokines, growth factors, direct cell-cell and cell-extracellular-matrix interactions, hormones, phorbol esters, UV radiation as well as viral and bacterial infections. It is predominantly regulated via MAPK-, PI3K- and NFκB- dependent transcription, but also via post-translational phosphorylation, mRNA turnover and protein stability [1][7][8]. Ultimately, these events determine specific transcriptional programs.
Accounting for ~10% of hematologic malignancies, Multiple Myeloma (MM) is characterized by the clonal expansion of malignant plasma cells (PCs) within the bone marrow (BM) and the abnormal increase of monoclonal paraprotein, leading to specific end-organ damage, including hypercalcemia, renal failure, anemia and lytic bone lesions (CRAB criteria)
[9]. The development of MM is initiated from a pre-malignant, asymptomatic stage called Monoclonal Gammopathy of Undetermined Significance (MGUS), and a more advanced pre-malignant, asymptomatic stage called Smoldering MM (SMM), due to cytogenetic alterations in post-germinal center (GC) PCs. During the evolution of MGUS or SMM into MM and ultimately PC leukemia (PCL), additional genetic aberrations as well as the supportive BM microenvironment play pivotal roles
[10][11]. The incidence of MGUS is >3% of the population over the age of 50, with a progression rate of 1% per year to MM; whereas SMM transforms to MM at a rate of ~10% per year during the first five years after diagnosis. As primary genetic events, approximately 40% of MM patients harbor trisomies of chromosomes, ~30% have immunoglobulin (Ig) heavy chain (IgH) translocations and ~15% have both trisomies and IgH translocations. The IgH locus is located on chromosome 14q32, and the translocations and genes affected include t(4;14)(p16;q32) (
FGFR3 and
MMSET), t(6;14)(p21;q32) (
CCND3), t(11;14)(q13;q32) (
CCND1), t(14;16)(q32;q23) (
c-MAF) and t(14;20)(q32;q11) (
MAFB). Secondary genetic events include gains and deletions of chromosomes, global hypomethylation, mutations and secondary translocations t(8;14)(q24;q32) (
MYC). Specifically, high-risk MM is characterized by the presence of gain of chromosome 1q, deletion of chromosome 17p (del(17p)), t(4;14), t(14;16), t(14;20) or p53 mutations
[9][12]. Despite therapeutic advances including the introduction of ImmunoModulatory Drugs (IMiDs), proteasome inhibitors (PIs), monoclonal antibodies and most recently selinexor, a Selective Inhibitor of Nuclear Export (SINE) that binds and inactivates exportin-1 (XPO1)
[13], the B Cell Maturation Antigen (BCMA) targeting antibody-drug-conjugate (ADC) belantamab-mafodotin
[14], and BCMA-directed CAR-T cells
[9], the management of MM remains challenging, mainly due to the development of drug resistance. Therefore, the identification of novel therapeutic targets and the development of derived anti-MM treatment strategies are urgently needed.
Our increasing knowledge of B cell differentiation and resultant generation of normal PCs have been fundamental to understand how these processes are deranged in MM cells
[15]. PCs that undergo IgH switch recombination home to the BM, where they occupy special survival niches, and become long-lived PCs
[16]. Besides their central role in many, if not all, physiologic processes, including PC differentiation, deregulation of AP-1 TFs has been implicated in solid and hematologic malignancies, including MM
[17]. Deregulation of TFs contributes to MM pathogenesis through: (1) direct TF modifications (e.g., mutations); (2) intrinsic genetic alterations or extrinsic stimuli within the BM microenvironment that trigger signaling pathway-mediated TF activation or inhibition; (3) epigenetic changes in DNA methylation, histone modifications and non-coding RNAs; and (4) TF dependency on prolonged oncogene activity (“oncogenic addiction”)
[11][18][19][20][21][22].
The present review article will comprehensively summarize our up-to-date knowledge on the critical role of AP-1 TFs in PC differentiation and MM pathophysiology. Moreover, we will discuss novel, rationally derived strategies to therapeutically target AP-1 TFs, including protein-protein and protein-DNA binding inhibitors, epigenetic modifiers and natural products.
2. AP-1 in Plasma Cell Biology
AP-1 TFs play a critical role in PC formation and function. Specific functions of selected AP-1 TF family members during PC differentiation will be discussed below (Table 1 and Figure 1). For details, please refer to the original article (10.3390/cancers13102326) [23].
3. AP-1 in Multiple Myeloma
Besides acting as critical regulators in PC differentiation, AP-1 TFs are emerging as “master regulators” of aberrant gene expression programs in MM. Below we will discuss functions of AP-1 TFs that have specifically been associated with MM pathogenesis during recent years, c-Maf and MafB, c-Jun, JunB, in particular. Whether Fra-1, Fra-2, B-ATF and other AP-1 family members are deregulated in MM cells is currently unknown and subject of our own and others’ ongoing research efforts (Table 1 and Figure 1). For details, please refer to the original article (10.3390/cancers13102326) [23].
Table 1. Function of AP-1 in plasma cell biology and multiple myeloma pathophysiology.
AP-1 Member |
Activity |
Mechanism |
References |
Plasma cell biology |
Fra-1 |
Suppresses B cell differentiation into PCs and decreases Ig production |
Inhibition of Prdm1/Blimp-1 expression by preventing binding of c-Fos to the promoter |
[24][25][26] |
Fra-2 |
Enhances B cell proliferation and differentiation at multiple stages |
Transcriptional induction of FOXO-1 and IRF-4 expression, and their downstream targets Ikaros, IL7Ra, Rag1/2 and Aiolos |
[27] |
B-ATF |
Essential for GC formation and effective CSR |
Downstream of FOXO-1, modulating the expression of Aicda/AID and GLTs from the Ig locus of B cells in the GC |
[28][29] |
Regulates B cell activation and GC response |
Binding of B-ATF containing AP-1 complexes and IRF-4 to the AICE motif of target genes |
[30][31] |
Multiple myeloma |
c-Maf MafB |
Overexpressed in MM |
Chromosomal translocation t(14;16), t(14;20) MMSET/MEK/ERK/AP-1 signaling sequelae |
[11][18][32] |
Promote MM cell proliferation, migration and invasion, survival, adhesion and pathological interactions with BMSC |
Regulation of cyclin D2, ARK5, DEPTOR, and integrin β7 expression |
[33][34][35] |
Confer resistance to PIs bortezomib and carfilzomib |
Abrogation of GSK3β-mediated proteasomal degradation of c-Maf and MafB |
[36][37] |
c-Jun |
Lower expression in primary MM cells compared to normal PCs |
Unknown |
[38] |
Upregulated in MM cells by adaphostin or bortezomib Inhibits proliferation and induces apoptosis |
Caspase-mediated c-Abl cleavage Upregulation of EGR-1 Upregulation of p53 |
[39][40][41][42] |
JunB |
BMSC- and IL-6- triggered upregulation in MM cells |
MEK/MAPK- and NFκB- dependent |
[43] |
Promotes MM cell proliferation |
Cell cycle regulation |
Protects MM cells against dexamethasone- and bortezomib- induced cell death |
Inhibition of apoptotic pathways |
Promotes MM BM angiogenesis |
Transcriptional regulation of angiogenic factors VEGF, VEGFB and IGF1 |
[44] |
Bone metabolism |
c-Fos |
Regulates OC differentiation (Block in OC differentiation in mice lacking c-Fos) |
Induced by RANKL and M-CSF Transcriptional regulation of Fra-1 and NFATc1 |
[45][46][47][48] |
Fra-1 |
Regulates OB activity and bone matrix formation (Mice overexpressing Fra-1 develop osteosclerosis) |
Regulation of bone matrix component production by OBs (osteocalcin, collagen1α2, and matrix Gla protein) |
[49][50] |
Fra-2 |
Regulates OB differentiation (Fra-2-overexpressing mice are osteosclerotic) |
Transcriptional regulation of osteocalcin and collagen1α2 |
[51] |
Controls OC survival and size (Increased size and numbers of OCs in Fra-2-deficient mice) |
Transcriptional induction of LIF via Fra-2: c-Jun heterodimers Modulation of LIF/LIF-receptor/PHD2/HIF1α signaling sequelae |
[52] |
JunB |
Regulates OB proliferation and differentiation (Mice lacking JunB are osteopenic) |
Cyclin D1 and cyclin A expression, and collagen1α2, osteocalcin and bone sialoprotein production |
[53] |
Regulates OC proliferation and differentiation |
Dimerization partner of c-Fos (?) |
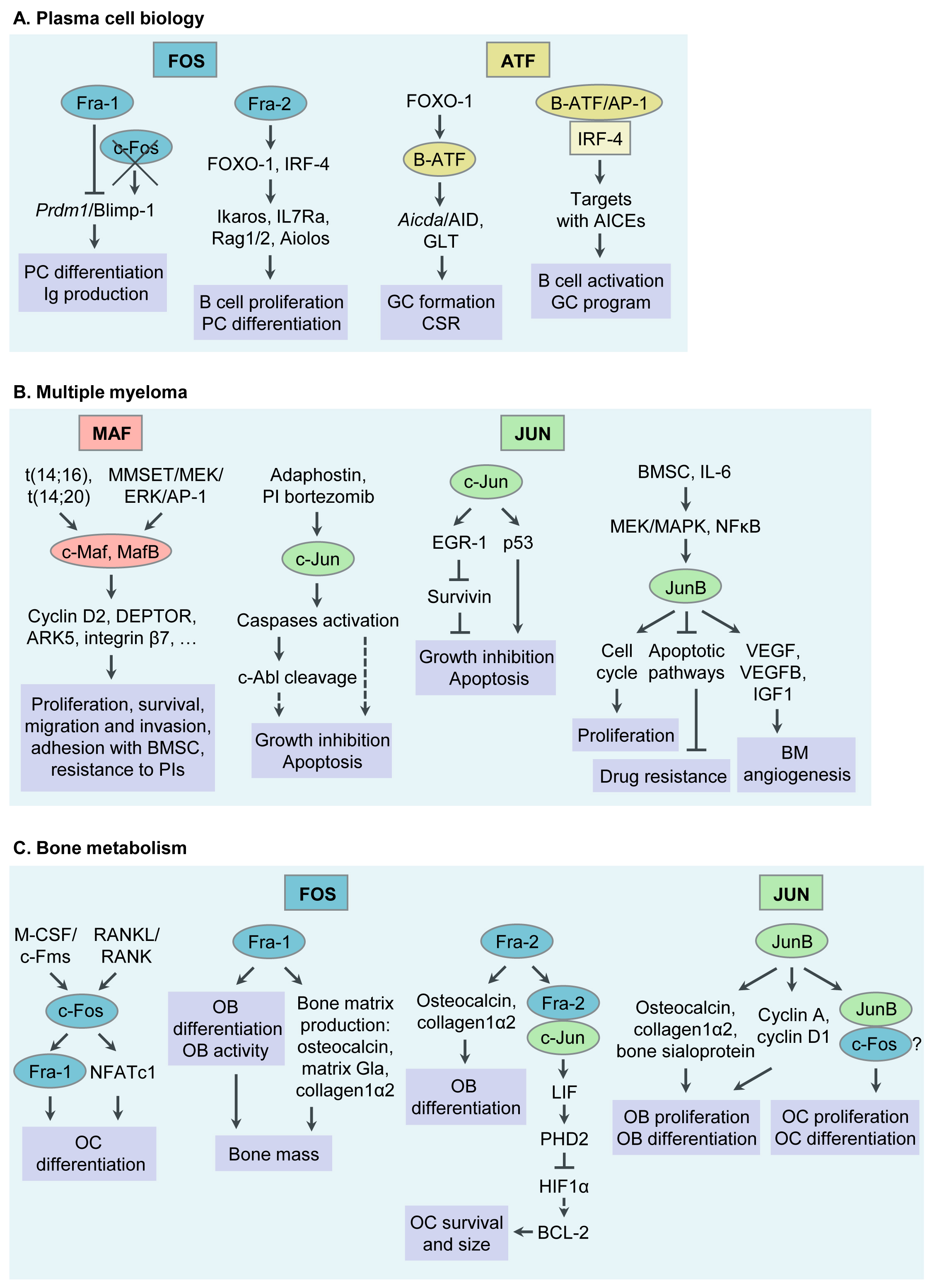
Figure 1. Functions of Activator Protein-1 (AP-1)/JUN, FOS, ATF and MAF transcription factor (TF) subfamily members in plasma cell (PC) biology, multiple myeloma (MM) pathophysiology, bone metabolism and MM associated bone disease. (A) Functions of AP-1 TFs in PC biology. (B) Functions of AP-1 TFs in MM pathogenesis. (C) Functions of AP-1 TFs in bone metabolism and MM associated bone disease. Ig, immunoglobulin; GC, germinal center; CSR, class switch recombination; AID, activation- induced cytidine deaminase; GLT, germline transcript; AICEs, AP-1-IRF composite elements; BM, bone marrow; BMSC, bone marrow stromal cell; PI, proteasome inhibitor; OC, osteoclast; RANKL, receptor activator of NFκB ligand; M-CSF, macrophage colony stimulating factor; NFAT, nuclear factor of activated T cells; LIF, leukaemia inhibitory factor; OB, osteoblast.
4. Targeting AP-1 TFs for MM Therapy
Accumulating evidence demonstrates a crucial role of deregulated AP-1 TFs in tumorigenesis in general, and MM in particular. AP-1 TFs therefore represent appealing therapeutic targets. However, TFs have been considered “undruggable” until recently due to their structural disorder (three-dimensional (3D) structure and architecture are very labile and dependent on TF interaction with functional proteins), their lack of tractable active sites (large protein-protein interfaces, lack of deep protein pockets) and their intracellular (often nuclear) localization. Nevertheless, with the progress of our understanding of the biochemical and biological properties of TFs, this paradigm does not hold true any longer. Indeed, members of the AP-1 family have emerged as worldwide actively pursued therapeutic targets, with a potentially high therapeutic index
[17][54][55][56]. In MM, our own and other studies suggest therapeutic strategies that inhibit c-Maf or JunB and induce c-Jun activity.
Besides inhibiting their expression (i.e., by siRNAs, miRNAs), novel approaches to target TFs in general, and AP-1 TFs in particular, include: (1) the disruption of either their interaction with functionally critical protein binding partners or; (2) their binding to the DNA (oligodeoxynucleotide decoys, pyrrole-imidazole polyamides or small molecules); (3) the modulation of their epigenetic binding through DNA methylation, histone methylation or modification; (4) the induction of proteasomal degradation of TFs by altering their ubiquitylation; as well as by utilizing PROteolysis-TArgeting Chimaeras (PROTACs) or Degronomids; (5) the inhibition of TF expression by modulating their regulators (i.e., MAPK- or NFκB-signaling molecules); (6) the use of reversible covalent drugs directed against non-conserved cysteines; and (7) the modulation of TF auto-inhibition. Moreover, disordered regions within TFs, which become structured upon interaction with binding partners (“coupled folding and binding”) may also represent attractive therapeutic targets. Indeed, these regions have a higher proportion of potential cavities and can more easily adjust to small molecules
[56][57][58][59][60]. Below we discuss some potential approaches to target AP-1 TFs in MM (Figure 2 and Table 2).
For details, please refer to the original article (
10.3390/cancers13102326)
[23].
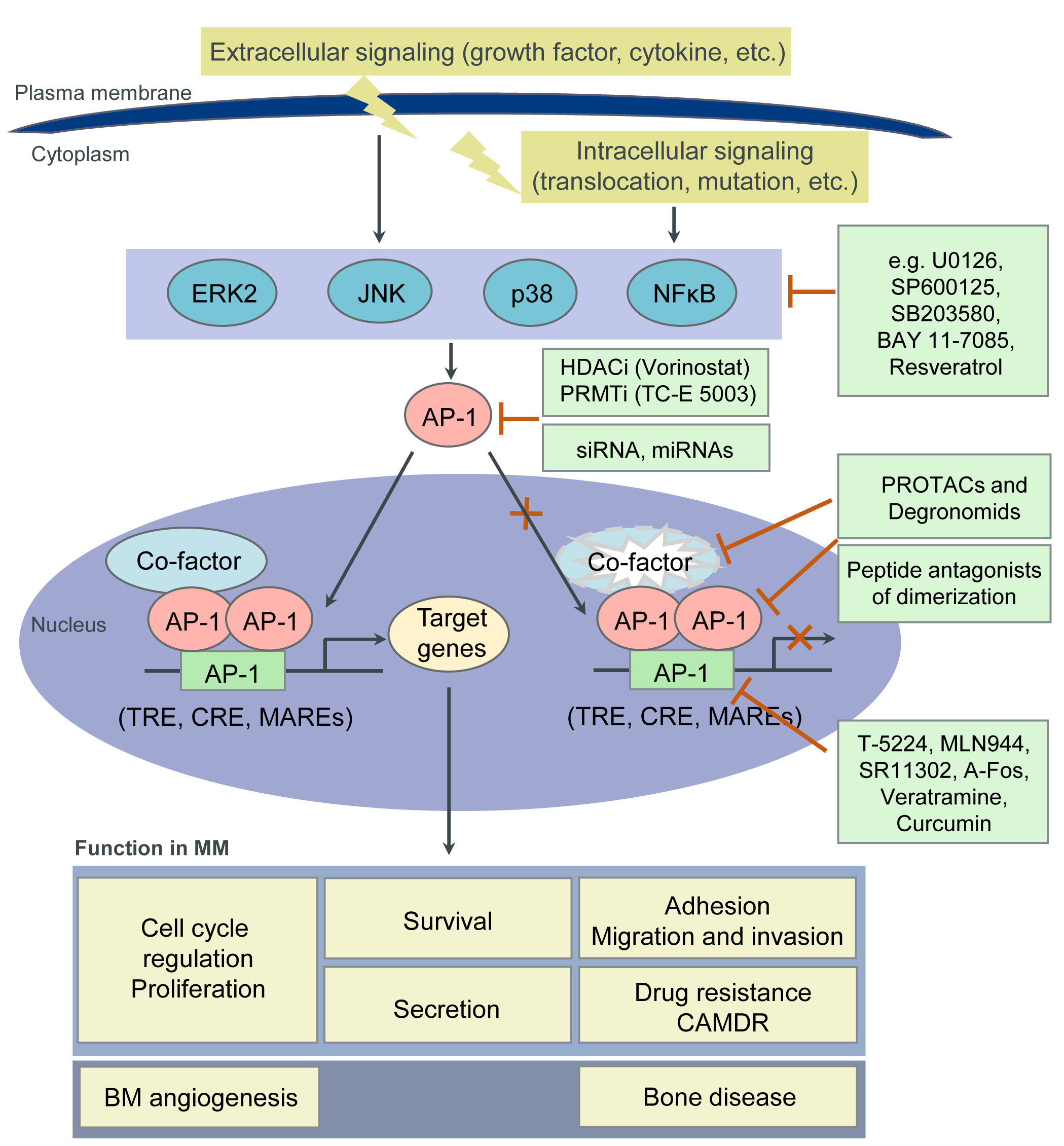
Figure 2. Pathophysiologic functions of AP-1 TFs in MM and derived therapeutic strategies. Intrinsic cellular (translocations, mutations) and intermittent extracellular environmental signals (e.g., growth factors, cytokines) trigger AP-1 TF activity via various signaling pathways including the extracellular-signal regulated kinase (ERK), JUN amino-terminal kinase (JNK), p38 and NFκB. Target genes of AP-1 TFs play a pivotal role in MM pathogenesis mediating tumor cell proliferation, adhesion, migration and invasion, apoptosis, survival and drug resistance as well as BM angiogenesis. Besides inhibiting TF expression by siRNAs or miRNAs, novel approaches to target TFs in general, and AP-1 TFs in particular, include: disrupting the interaction of TFs with either functionally critical protein binding partners (e.g., by peptide antagonists of dimerization) or the DNA (by oligodeoxynucleotide decoys, pyrrole-imidazole polyamides or small molecules); modulating the epigenetic events through DNA methylation, histone methylation or modification (e.g., by histone deacetyltransferases inhibitors (HDACi) or protein arginine methyltransferases inhibitors (PRMTi)); inducing proteasomal degradation of TFs by altering their ubiquitylation, as well as by utilizing PROteolysis-TArgeting Chimaeras (PROTACs) or Degronomids; and inhibiting TF expression by modulating their regulators (i.e., MAPK- or NFκB- signaling molecules).
Table 2. Potential strategies to target AP-1 and candidate inhibitors.
Strategies |
Inhibitors |
Targets |
References |
Inhibition of protein-protein interactions |
Peptidic inhibitors of c-Maf dimerization |
Leucine zipper motif of c-Maf |
[61] |
Peptide antagonists of c-Jun dimerization |
Leucine zipper motif of c-Jun |
[62][63][64][65] |
Peptide antagonists of c-Jun: c-Fos dimerization |
Leucine zipper motif of c-Jun or c-Fos |
[66][67] |
Leucine zipper peptide (Superzipper) |
Leucine zipper dimerization domains of both c-Jun and c-Fos |
[68] |
Inhibition of protein- DNA binding |
T-5224 |
bZIP domain of c-Fos/AP-1 -DNA complex |
[69][70] |
MLN944 (XR5944) |
TRE |
[71] |
SR11302 |
TRE |
[72][73] |
Dominant negative peptide A-Fos |
bZIP domain of c-Jun |
[74] |
Regulation of epigenetic events |
Valproic acid (VPA) Vorinostat (SAHA) Trichostatin A (TSA) LBH589 |
HDAC (Transcriptional suppression of c-Jun and Fra-1 expression) |
[75] |
TC-E 5003 (TC-E) |
PRMT (Suppression of c-Jun expression and nuclear translocation) |
[76] |
Natural products |
Curcumin |
Suppression of c-Fos and c-Jun expression and their binding to DNA |
[77] |
Resveratrol |
Suppression of c-Fos and c-Jun expression and AP-1 activity |
[78] |
Veratramine |
TRE |
[79] |