1. Introduction
The gastrointestinal tract (GIT) microbiota plays a pivotal role in influencing critical factors such as feed efficiency [1] , growth performance [2] and susceptibility to diseases [3] in pigs. Interventions targeting improvements in the GIT microbiota through dietary adjustments have proven to be notably effective across all developmental stages of pigs, resulting in enhancements in average daily gain, food conversion ratio (FCR), and overall animal health [4][5][6]. A probiotic, defined as a "live microorganism which, when administered in adequate amounts, confers a health benefit on the host," offers a promising avenue for modulating the GIT microbiota. These health benefits can manifest through various mechanisms, encompassing both direct actions of the probiotic itself and indirect effects mediated by the production of beneficial metabolites and secretions.
2. Mechanisms of Action of Probiotics
There are numerous mechanisms through which probiotics can confer health benefits to the host, and these can be both direct, via the probiotic itself, and/or indirect, via the production of beneficial metabolites and secretions (Figure 1). The following is a description of the beneficial modes of action depicted in Figure 1.
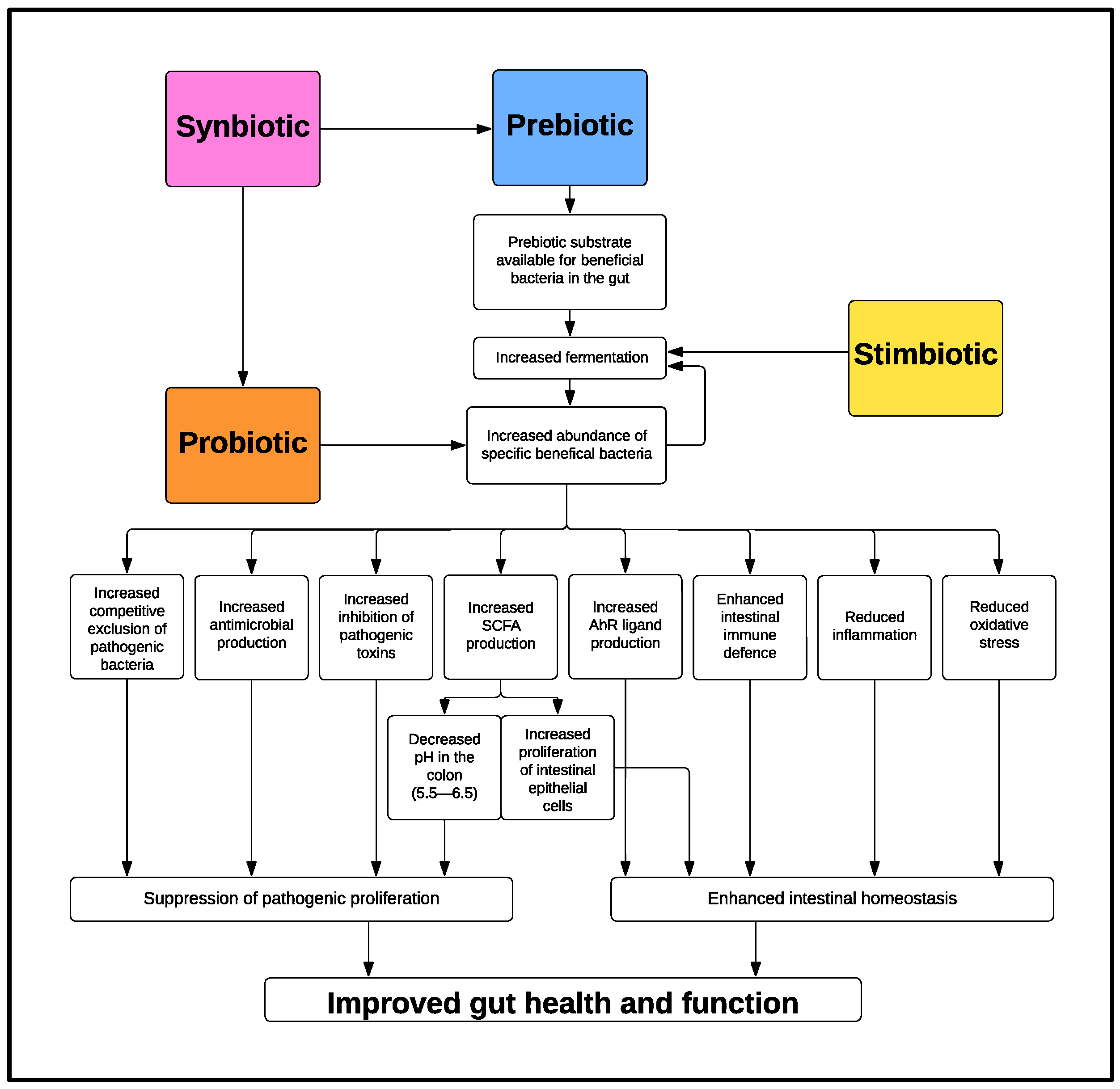
Figure 1. Mechanism of Action of Probiotics, Prebiotics, Synbiotics and Stimbiotics.
2.1. Competitive Exclusion of Pathogenic Bacteria
The initial stages of intestinal infection involve the pathogen adhering to the intestinal mucosal surfaces. Certain probiotic bacteria compete with pathogenic bacteria for these crucial adhesion sites. Probiotics disrupt pathogen adhesion using several mechanisms, including the formation of an auto-aggregation barrier, which occurs when bacterial cells adhere to each other
[7][8]; competitive displacement, which occurs when probiotic bacteria out-compete pathogens for adhesion sites
[7][9]; and co-aggregation, which occurs when probiotic bacteria attach with pathogens via specific molecules and prevent intestinal surface colonization
[8][10][11]. Competitive displacement occurs when the probiotic outcompetes the pathogen by hindering its attachment, competing for nutrients, and reducing the fitness of competing bacteria via its antimicrobial factors
[9]. The ability of a probiotic to adhere to the mucosa varies depending on the strain, its location in the intestine, and existing competition for adhesion sites
[8][12][13]. The majority of strains exhibit significant pathogenic adhesion inhibitory effects; however, certain strains, such as
Lactobacillus rhamnosus, are more effective than others
[12][14][15].
As mentioned, one method for competitive displacement of pathogenic bacteria by probiotic bacteria involves competition for nutrients in the GIT. The regular supply of nutrients into the GIT means there are generally sufficient quantities to support the growth of most bacteria; however, for competitive exclusion to occur, there need only be competition for a single essential nutrient. For example,
Salmonella Typhimurium is a pathogen that thrives in an inflamed GIT. It has adapted to acquire essential micronutrient metals in limited availability
[16]. Administering the probiotic
Escherichia coli Nissle, which assimilates iron in a similar mechanism to
Salmonella Typhimurium, limits the ability of the pathogen to colonize the GIT
[17][18]. The probiotics
Lactobacillus amylovorus and
Lactobacillus mucosae do not suppress the growth of salmonella at any location in the GIT, as they cannot outcompete
Salmonella Typhimurium for iron
[17]. These studies highlight the process of competitive exclusion via competition for a single crucial nutrient.
2.2. Antimicrobial Production
Another desirable property of a probiotic strain is its ability to limit the growth of pathogenic bacteria via metabolite secretions. The supernatant of the probiotic can contain a mixture of metabolite secretions, such as organic acids and bacteriocins, that inhibit the growth of pathogens. The production of organic acids reduces the pH of the surrounding environment, which favours the growth of beneficial microbes while inhibiting a range of harmful microbes
[19][20]. Bacteriocins are specialized antimicrobial proteins produced by certain strains to inhibit pathogen growth.
Lactobacillus and
Bacillus species, for example, produce a range of antimicrobial substances, such as bacteriocins and antifungal metabolites, that reduce the growth of pathogenic bacteria
[14][21][22][23][24].
In addition to reducing the pH and producing antimicrobial molecules, certain probiotics can modulate the expression of virulence genes in pathogenic bacteria. Quorum sensing is a process of cell-to-cell communication that allows bacteria to regulate their gene expression in response to the cell population density
[25][26]. In response to high cell densities, bacteria produce signalling molecules known as autoinducers. Pathogenic bacteria control the expression of their virulence genes by producing these autoinducers. Certain probiotics can secrete enzymes, known as quorum quenching enzymes, that degrade these autoinducers that signal increases in the expression of virulence genes in pathogenic bacteria
[2][27][28][29]. Through quorum quenching, certain probiotic bacteria can control the proliferation of pathogenic bacteria and significantly modulate the GIT microbiota
[29].
2.3. Inhibiting Pathogenic Toxins
Toxins secreted by pathogenic bacteria, such as Shiga toxins and enterotoxins, can directly lead to intestinal dysfunction, including loss of water and electrolytes. These toxins can negatively affect barrier function by a number of different mechanisms, including manipulation of the tight junction and cytoskeletal proteins
[1][3]; passing through the enterocytes (via endocytosis, internalization, or the oligomerization of toxins) to form pores in the intestinal cell membranes
[4]; and altering the isotonic stability in the cells of the intestine
[5]. Certain probiotics can neutralize or inhibit toxins produced by pathogenic bacteria.
Lactobacillus zeae inhibits ETEC enterotoxin production
[6], while
Lactobacillus kefir can reduce the cytopathic effect of
Clostridium difficile [30]. Furthermore,
Lactobacillus,
Pediococcus, and
Bifidobacterium strains downregulate the expression of the
Escherichia coli toxin, Shiga toxin 2 (
STX2A) gene, driven by the production of organic acids by the probiotic strains and subsequent decrease in pH
[31].
2.4. Short-Chain Fatty Acid Production
Short-chain fatty acids, mainly acetate, propionate, and butyrate, are organic acids that are predominantly produced in the GIT through anaerobic bacterial fermentation of undigested dietary fibre. The SCFA have numerous beneficial effects, including promoting GIT barrier function, providing an energy source for epithelial cells, maintaining immune homeostasis, and modulating the GIT microbiota
[32][33]. The quantity and type of SCFA produced depends on the physical and chemical properties of the substrate available to the probiotic coupled with the existing microbial composition of the GIT
[34]. Increasing concentrations of SCFA reduces the pH, creating an unfavourable growth environment for pathogenic bacteria
[19][20]. Butyrate is also a primary energy source for epithelial cells and has a crucial role in supporting colonic cell proliferation and intestinal growth
[35][36]. The SCFA support multiple aspects of the GIT immune system, including improved intestinal barrier function
[37], increased expression of β-defensins and cathelicidins
[38], and immune responses, such as maintaining T cell homeostasis
[39] and accelerating the differentiation of naïve T cells to Treg cells
[40]. The regulation of the immune cells by SCFA is reviewed in detail by Liu et al.
[41]. This immune modification by SFCA is suggested to be mediated via cell surface G protein-coupled receptors and inhibition of histone deacetylase and potential activation of histone acyltransferase
[39][42][43]. The modification of epigenetic landmarks, such as histone deacetylase and acyltransferase, modulate the host’s gene expression. The current understanding and knowledge of the epigenetic effects of SCFA on immune system cells has been recently reviewed
[44][45].
2.5. Microbiota Derived Aryl Hydrocarbon Receptor Ligands
Aryl hydrocarbon receptor ligands are another important and beneficial metabolite group
[46]. The AhR is expressed in both immune and nonimmune cells of the GIT and has emerged as a key player in the regulation of intestinal homeostasis (as reviewed in
[47]). There are numerous sources of endogenous and exogenous AhR ligands, one endogenous source being the GIT microbiota itself. These AhR ligands are typically produced by the microbiota via the metabolism of tryptophan
[46]. The microbiota produces a variety of AhR ligands, which are typically indole derivatives such as indole-3 ethanol (IE), indole-3 pyruvate
[48], indole-3 aldehyde (I3A), and tryptamine (TA)
[49]. The SCFA, butyrate, has also been reported to stimulate AhR activity
[50]. Stimulation of AhR by these ligands is known to enhance intestinal barrier function
[51][52] and reduce inflammation
[53]. It is suggested that these effects are mediated via IL-22, a cytokine involved in multiple aspects of intestinal barrier function and intestinal integrity
[54][55]. Recently, there has been an increased focus on the concentration of tryptophan-derived AhR ligands in the digesta and faeces as potential markers for improved gut health
[56][57].
Interestingly, in colitis-induced mice, intestinal inflammation is attenuated following inoculation with three
Lactobacillus strains capable of metabolizing tryptophan or by treatment with an AhR agonist
[58]. Similarly, I3A, an indole derivative that is produced via tryptophan metabolism by
Lactobacilli's tryptophan metabolism, restores IL-22 production and ameliorates colitis when administered to colitis-induced mice
[54]. Supplementation with the indole derivative, indole-3-carboxaldehyde, to weaned pigs has no effect on intestinal barrier function and morphology or growth performance in the postweaning phase but increases alpha diversity in the colon and increases jejunal, ileal and colonic indexes while also upregulating the expression of proliferating cell nuclear antigen in the intestine
[59]. Supplementing co-cultures of
Lactobacillus acidophilus and
Bacillus subtilis to postweaned pigs has beneficial effects on performance and a number of GIT health parameters, but more precisely, it leads to the upregulation of the protein expression of IL-22 and AhR
[60]. The supplementation of pectin, a prebiotic substrate, to pigs in the postweaning phase increases the abundance of
Lactococcus and
Enterococcus abundance, increases tryptophan-metabolite concentration, increases the expression of
AHR,
IL-22, and other AhR-IL22 pathway genes, improves intestinal integrity and reduces inflammation
[57]. These studies highlight the potential of utilizing probiotics with strong tryptophan metabolizing and AhR activating capabilities as a therapeutic strategy to enhance host GIT health. To determine their efficiency, further investigation into probiotics with these capabilities is warranted.
2.6. Enhancing Intestinal Immune Defence
The intestine is continuously exposed to foreign substances, and its innate immune response and interaction with the adaptive immune system is crucial to ensuring optimal health. To protect the body from foreign antigens, the intestine has a number of innate defence mechanisms, including: (1) epithelial barrier integrity, (2) host defence peptides and secretory immunoglobulin A, and (3) mucus layer. The main environmental factors that can affect the functioning of these defence mechanisms include toxins
[61], pathogens
[62], and stress
[63]. Probiotics and their metabolites are associated with the enhancement of multiple different aspects of the intestinal immune defence, as follows:
- (1)
-
Epithelial barrier integrity
The intestinal epithelial layer serves as a barrier between the luminal environment and the body, regulating the uptake of essential nutrients and water while preventing the entry of foreign antigens into the paracellular area. The integrity and permeability of the barrier are maintained by intracellular adhesion complexes, known as tight junctions, that contain multiple transmembrane proteins. Dysregulation of these proteins can increase gut permeability, leaving the host susceptible to infection. Many pathogenic bacteria produce enterotoxins, which modulate the epithelial barrier function through the manipulation of tight junctions and cytoskeletal cell components
[1][3][64]. Heat stress and the stress of weaning are associated with reduced intestinal barrier integrity
[65][66]. Certain probiotic strains, such as
Lactobacillus plantarum,
Lactobacillus reuteri, and
Lactobacillus casei, increase tight junction gene expression and protein abundance and thereby enhance intestinal barrier function
[67][68][69].
Transepithelial electrical resistance (TEER) is a measurable parameter associated with barrier function of in vitro models
[70]. Probiotic strains have been demonstrated to maintain and improve TEER
[68][71]. Importantly, probiotics help to maintain TEER in the presence of pathogenic bacteria by enhancing the expression of tight junction proteins
[68][72][73]. This is also evident in vivo;
Lactobacillus casei supplementation increased tight junction proteins and was highlighted as an effective preventative treatment against the adverse effects of
Escherichia coli, specifically its effects on tight junction proteins
[69]. It has been suggested that the mechanism for improved barrier function is mediated by signalling via the pattern receptor, Toll-like receptor-2 (TLR2)
[74][75]. Gastric infusion of SCFA increases mRNA abundance of Occludin (
OCLN) and Claudin-1 (
CLDN1) in weaned piglets, highlighting SCFA as the key mechanism through which probiotics improve barrier function
[37], and as discussed previously, AhR ligands may also contribute
[51]. These studies highlight the cumulative effect of probiotics and their metabolites on improving gut barrier function.
- (2)
-
Host defence peptides and secretory immunoglobulin A
Host defence peptides (HDPs) are antimicrobial peptides that are part of the innate immune system, produced in various body cells and tissues
[76]. There are two major categories of HDPs in pigs: defensins and cathelicidins
[77]. Enhancing the secretion of HDPs can improve the pig’s intestinal innate immune response due to their antimicrobial activity
[78][79]. Several probiotics, including multiple strains of
Lactobacillus, increase the expression of HDPs without triggering an inflammatory response
[67][80][81][82][83]. To date, the exact mode of action is unclear, but with regard to
Lactobacillus plantarum it is suggested that TLR2 is involved in the subsequent upregulation of HDPs
[82]. The increased production of SCFA associated with probiotic supplementation may also play a role
[84]. The SCFA, especially butyrate, are known to increase the secretion of HDPs
[38]. Another secretory product that plays a role in mucosal defence is secretory IgA (sIgA). In the intestine, sIgA is secreted into the mucus, where it binds to pathogens and toxins, preventing their adhesion to the epithelium in a process referred to as immune exclusion while also promoting immune tolerance and gut homeostasis (as reviewed in
[85]). Interestingly, supplementation with probiotic
Lactobacilli strains leads to an increase in sIgA in piglet faeces, indicating that the probiotic enhances the secretion of sIgA in the intestine
[86][87][88].
- (3)
-
Mucus layer
Goblet cells are specialized secretory cells that populate the GIT and are responsible for the production of the mucin glycoproteins as the principal component of the protective mucus barrier. In the small intestine, there is a single unattached “loose” mucus layer; however, in the colon, there are two distinct layers of mucus, comprised of an attached “thick” inner layer and an unattached “loose” outer layer
[89][90]. The single mucus layer of the small intestine enables the absorption of nutrients while limiting bacterial contact with the epithelium
[90][91][92]. In the large intestine, the inner layer is impermeable to bacteria, preventing them from overactivating the immune sensing and responsive cells in this region and triggering inflammation
[89][93][94]. In contrast, the outer mucus layer of the colon is a habitat for microbes called mucus-associated microbes, which utilize the mucin glycans as attachment sites
[95][96].
Some pathogens secrete enzymes that cleave the mucins, and this is a key aspect to their pathogenicity
[97]. Related to this, other pathogenic microbes rely on the breakdown of the mucus layer by other bacteria to proliferate and then opportunistically infect the host
[98]. The mucus layer can become impaired during periods of intestinal dysfunction, such as at weaning time, increasing the risk of exposure to pathogenic microorganisms
[99]. To this extent, stabilizing the mucus layer by promoting optimal goblet cell numbers/activity and mucus secretion is beneficial to host health and can potentially reduce the risk of infection by pathogens. Probiotic supplementation to pigs has been shown to enhance goblet cell numbers and mucus expression in the intestine
[100][101][102]. For example, supplementing two-week-old pigs with a blend of lactic acid bacteria and yeast increases the number of goblet cells in the large intestine
[103]. Pigs challenged with ETEC have reduced goblet cell numbers in the intestine; however, pretreatment with
Bacilluslicheniformis and
Bacillussubtilis offsets this decrease in ETEC-challenged pigs
[101]. Pretreatment with a moderate dose of
Bacilluslicheniformis and
Bacillussubtilis increases
MUC2 expression in the intestine of both ETEC-challenged pigs and unchallenged control pigs
[101]. These studies highlight the potential for probiotics to enhance goblet cell numbers and mucin secretion, thereby stabilizing the mucus layer of the intestine.
2.7 Reducing Inflammation
Cytokines are small signalling proteins produced by a wide range of immune and intestinal epithelial cells. Cytokines can be pro- or anti-inflammatory and play a key regulatory role in the immune and inflammatory response. A balance between these classes of cytokines is a crucial aspect to obtaining a regulated immune response in the gut, as uncontrolled inflammation has negative effects on the integrity and functioning of the GIT barrier
[104]. In particular, increased and unregulated production of proinflammatory cytokines such as TNF-α, IFN-γ, and IL-6 are associated with reduced barrier integrity
[68][105]. A number of probiotic species have been shown to restore the balance of inflammation in in vitro models of the gut. The addition of
Lactobacillus reuteri can offset stimulated inflammation by maintaining barrier integrity in an LPS-stimulated porcine jejunal epithelial cell line (IPEC-J2) and also reduces the expression of both TNF-α (
TNF) and IL-6 (
IL6)
[68]. Pretreatment of intestinal porcine cells with the probiotic
Lactobacillus plantarum decreases the expression of pro-inflammatory cytokines IL-8 (
CXCL8) and TNF-α (
TNF) when stimulated with ETEC K88
[62].
Lactobacillus amylovorus suppresses TLR4 signalling caused by
Escherichia coli [106]. These studies suggest that many probiotics can rebalance the overexpression of pro-inflammatory cytokines caused by pathogenic immune stimulation.
2.8 Reducing Oxidative Stress
Oxidative stress is caused by an imbalance between oxidants and antioxidants in favour of oxidants, also referred to as reactive oxygen species (ROS), which in turn can lead to cell and tissue damage
[107]. There are a number of developmental stages, including gestation, lactation, and weaning, where the pig is normally subjected to oxidative stress. Environmental factors, including mycotoxins in feed, social change and disease, can also induce oxidative stress
[108]. Oxidative stress is typically accompanied by a reduction in average daily gain (ADG) and a reduction in average daily feed intake (ADFI)
[109]. Intestinal oxidative stress can reduce GIT barrier integrity and dysregulate cell homeostasis, leading to erosion of the intestinal villi and significant losses in intestinal enzyme activity and absorptive function
[109]. Oxidative stress and inflammation are interconnected in a complex interplay where one can easily be induced by another (as reviewed in
[110]).
Interestingly, probiotics and their metabolites can help reduce oxidative stress and alleviate the negative issues associated with it
[111][112][113]. The culture supernatant, intact cells and intracellular free extracts of
Bifibacterium animalis 01 all have strong antioxidant activity in vitro
[111]. In vivo, the inclusion of multi-species probiotics, including
Lactobacillus acidophilus,
Lactobacillus casei,
Bifidobacterium thermophilum, and
Enterococcus faecium to pigs in the postweaning phase reduces intestinal oxidative stress
[112]. The inclusion of
Lactobacillus salivarius or
Lactobacillus plantarum in the diet of pigs postweaning improves the antioxidant capacity of the pig, evident from markers present in both the blood and intestine
[113][114].
Bacillusamyloliquefaciens supplementation reduces the oxidative stress marker malondialdehyde (MDA), in the intestine of finisher pigs while increasing the expression of antioxidant enzymes
[115].
Lactobacillus casei supplementation alleviates the spike in MDA in the liver of postweaned pigs challenged with LPS, while also increasing levels of the antioxidant enzyme superoxide dismutase (SOD)
[116]. The beneficial effects observed in these studies suggest the potential of probiotics as therapeutic agents targeting the reduction in oxidative stress in the pig and may be particularly useful at specific time points, including weaning, gestation, and lactation.
2.9 Other Potential Beneficial Effects: Vitamins and Enzymes
Certain bacteria in the GIT microbiota, such as
Bifidobacteria, supply vitamins to the host by de nova synthesis. Most water-soluble B vitamins, such as vitamin B-6, vitamin B-12, biotin, riboflavin, thiamine, and vitamin K are all produced by the microbiota
[117]. While the production of vitamins by the microbiota has long been established, the mechanisms of absorption and bioavailability of these vitamins to the pig are less well known. Specific transporters for the different water-soluble B vitamins have been identified in the colon of humans and mice
[118][119][120][121]; however, analogous studies are limited in pigs. Branner and Roth-Maier documented aspects of synthesis and absorption of several B vitamins in grower pigs and reported that: thiamine and biotin were synthesized in the colon, but the degree of absorption was unclear as levels were greater in the faeces than precaecal digesta; riboflavin and pantothenic acid were absorbed and utilized in the colon, as there were greater levels in the precaecal digesta than in the faeces
[122]. While this study confirms the synthesis of vitamins by the GIT microbiota and vitamin utilization, at least for certain vitamins, the extent of the absorption of these vitamins remains unclear. Certain bacteria utilize either dietary or B vitamins produced by other bacteria, and it is suggested that there may be competition between the host and the intestinal bacteria for these B vitamins
[123]. The production of vitamin K and B vitamins is often cited in the literature as a beneficial feature of the microbiota; however, the extent of the availability and utilization of these vitamins in pigs has not been established fully.
In addition to vitamin production, probiotics, such as
Bacillus and
Lactobacillus strains, produce extracellular enzymes that break down undigested food in the GIT
[124][125][126][127]. Hence, with regard to pig nutrition, additional nutrients are made available in this way, contributing to improved feed utilization
3. Conclusion
In conclusion, probiotics showcase a wide array of mechanisms, including competitive exclusion, antimicrobial production, and toxin inhibition, underscoring their efficacy against pathogenic bacteria. Additionally, the beneficial effects of microbiota-derived metabolites such as short-chain fatty acid and the AhR ligands can fortify immune defences, mitigate inflammation, and reduce oxidative stress. In essence, the diverse and intricate modes of action outlined underscore the significance of probiotics as agents capable of positively influencing various aspects of gastrointestinal health. Further research and exploration into specific strains and their applications are warranted to discover the full potential of probiotics in promoting health and performance.