Pathological cardiac hypertrophy is a key risk factor for the development of heart failure and predisposes individuals to cardiac arrhythmia and sudden death. While physiological cardiac hypertrophy is adaptive, hypertrophy resulting from conditions comprising hypertension, aortic stenosis, or genetic mutations, such as hypertrophic cardiomyopathy, is maladaptive. Prolonged cardiovascular stress causes cardiomyocytes and non-myocardial cells to enter an activated state releasing numerous pro-hypertrophic, pro-fibrotic, and pro-inflammatory mediators such as vasoactive hormones, growth factors, and cytokines, i.e., commencing signaling events that collectively cause cardiac hypertrophy. Fibrotic remodeling is mediated by cardiac fibroblasts as the central players, but also endothelial cells and resident and infiltrating immune cells enhance these processes. Many of these hypertrophic mediators are now being integrated into computational models that provide system-level insights and will help to translate our knowledge into new pharmacological targets.
1. General Introduction
Myocardial remodeling associated with cardiac hypertrophy is one of the critical causes in the development of heart failure. The pathogenesis of heart dysfunction is one of the primary causes of morbidity and mortality in elderly people
[1].
Cardiac hypertrophy is the most frequently compensatory or adaptive process to numerous physiological or pathological conditions
[2]. Hypertrophic enlargement is characterized by an increase in the cell size of cardiomyocytes. The heart can dynamically change its muscle mass to cope with the stimuli of development, physiological conditions of exercise and pregnancy, or pathological disease stimuli
[3]. Increased workload as a consequence of volume or pressure overload due to pathological or physiological stimuli increases tension on the cardiac walls of the heart chambers
[4][5]. This ultimately triggers stress signals released by different cell types of the microenvironment to compensate for the wall tension increase, resulting in a hypertrophic growth response
[4][5]. Individual cardiomyocytes can increase in length and/or width in response to hypertrophic stimuli depending on the intracellular signaling cascades involved
[6][7][8].
Hypertrophy, or the enlargement of individual muscle fibers, is the primary mechanism by which skeletal muscle mass increases during postnatal development. A similar process may be induced in adult skeletal muscle in response to contractile activity, satellite cell proliferation and fusion, which increases the number of myonuclei. This event may also play a role in muscle growth during early but not late stages of postnatal development and in some forms of muscle hypertrophy
[9]. Likewise, the number of endothelium and mesenchymal cells can also increase from birth through early adulthood, but on the other hand the entire complement of cardiomyocytes is created during pregnancy and remains nearly constant throughout the human lifespan.
2. An Interplay of Different Cells in Hypertrophic Remodeling
The heart consists of various cell types, including myocytes, endothelial cells, fibroblasts, vascular smooth muscle cells, sympathetic neurons, and immune cells, which collectively account for a synchronized cardiac function
[10][11]. However, it has been shown that owing to their enormous size, cardiomyocytes in particular account for the majority of heart mass, increase in size and reprogram transcription in the process of cardiac hypertrophy
[2][12]. Communications between cardiomyocytes and non-myocytes lead to the secretion of bioactive mediators, which operate in an autocrine and paracrine manner. This is followed by microenvironmental stimulation of different cell types and the activation of various signaling pathways within the cells (
Figure 1 and
Figure 2)
[13][14]. Altogether these complex processes result in cardiomyocyte hypertrophy, fibroblast hyperplasia, interstitial tissue composition changes, and remodeling of the ventricular chambers
[15].
2.1. Fibroblast Remodeling
Pressure overload triggers resident cardiac fibroblasts originating from the epicardium and endocardium to undergo rapid expansion and activation, rather than previously reported hematopoietic precursor-derived fibroblasts or endothelial-to-mesenchymal transition (EndMT) as a contributing source (
Figure 1 and
Figure 3)
[16][17]. Despite this, the exact origins of cardiac fibroblasts as well as the delineation of their characteristics and plasticity remain a field of current investigation and controversy
[18]. Like cardiomyocytes, fibroblasts respond to external stress stimuli, but in a slightly different manner. Mechanical stress promotes fibroblast differentiation to a myofibroblast-like phenotype (
Figure 1 and
Figure 3)
[19][20], which has been shown to develop from tissue-derived fibroblasts rather than endothelial or smooth muscle cells
[17].
2.2. Endothelial Cell Activation
In response to pressure overload, cardiac endothelial cells, similar to cardiac fibroblasts, are capable of changing their phenotype (
Figure 1). It has been reported that endothelial cells can undergo an EndMT, differentiate into myofibroblast-like cells, and thereby contribute to cardiac fibrosis
[21]. Others outlined that EndMT recruits circulating hematopoietic progenitors to the heart thereby generating significant numbers of cardiac fibroblasts (reviewed in
[22]) but also their origin from tissue-resident fibroblasts is being discussed
[16][17]. Altogether, left ventricular myocardial tissue of end-stage cardiac failure patients revealed dramatically increased expression levels of EndMT-related genes
[23], indicating the need for further investigation to clarify the exact contribution of EndMT.
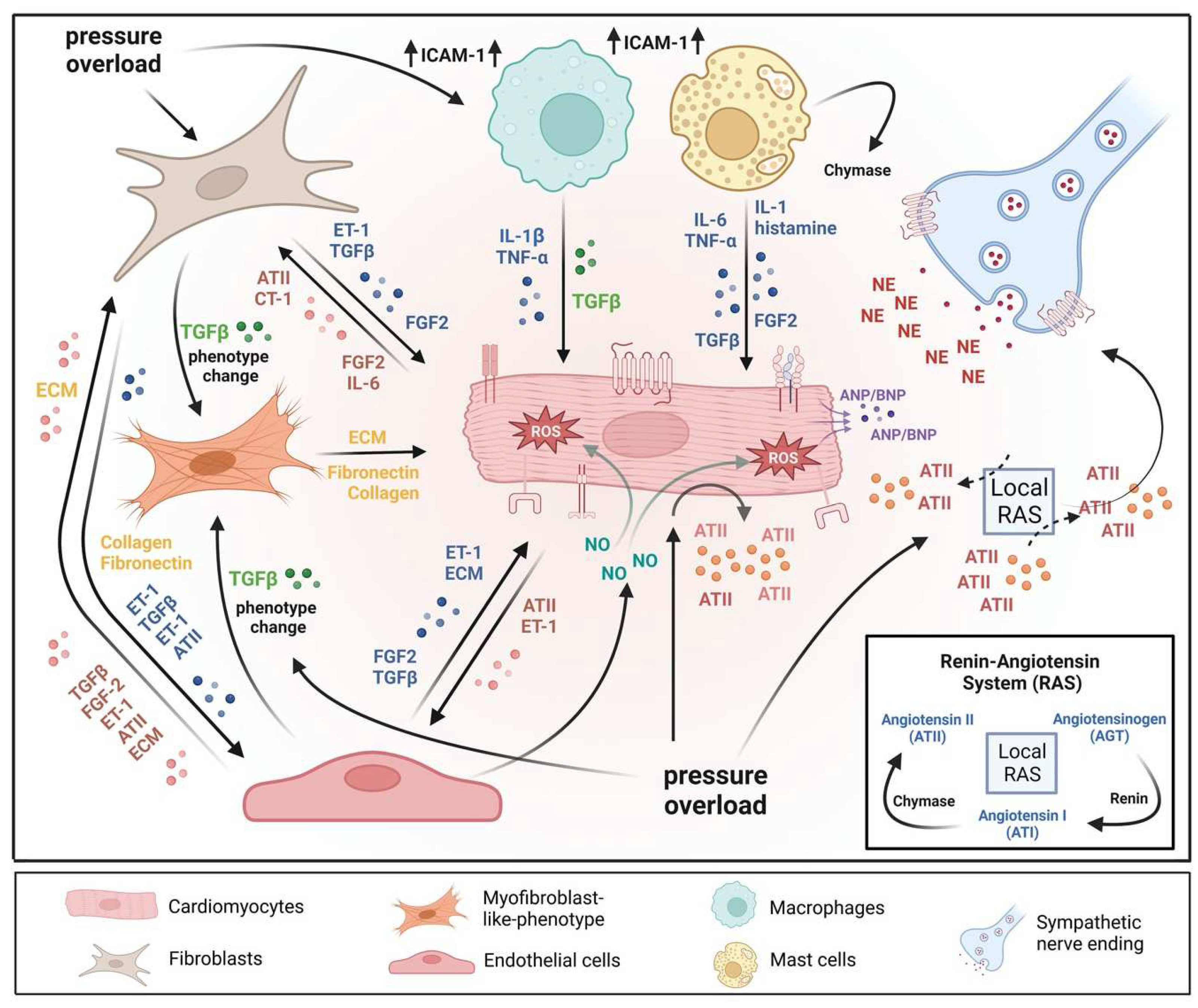
Figure 1. A microenvironmental model of pressure overload-induced cardiac hypertrophy. The model also illustrates multiple cell types’ substantial roles and reciprocal interactions in the myocardium. In response to pressure overload cardiomyocyte and non-myocardial cells are transformed into an “activated state”, releasing numerous pro-hypertrophic, pro-fibrotic, and pro-inflammatory mediators. In addition, vasoactive hormones, various growth factors, cytokines, and the local renin-angiotensin system (RAS) act in an autocrine and/or paracrine mode. Collectively, the above-mentioned mechanisms orchestrate effects that contribute to pathological remodeling processes leading to cardiac hypertrophy, fibrosis, and inflammation. AT II: angiotensin II; CT-1: cardiotrophin-1; ECM: extracellular matrix; ET-1: endothelin-1; FGF-2: fibroblast growth factor-2; ICAM-1: intercellular adhesion molecule-1; IL-1: interleukin-1; IL-6: interleukin-6; NE: norepinephrine; TGF-ß: transforming growth factor-ß; TNFα: tumor necrosis factor-α.
Major factors secreted by cardiac endothelial cells comprise nitric oxide (NO), endothelin 1 (ET-1), prostaglandin I2 (PI2), and angiotensin II (AT-II), which directly influence cardiac metabolism, growth, contractile performance, and rhythmicity of the adult heart
[24]. In response to various stimuli, activated endothelial cells express adhesion molecules, including intercellular adhesion molecule-1 (ICAM-1) and vascular cell adhesion molecule-1 (VCAM), which attract and further promote the infiltration of immune cells into the myocardium (
Figure 1). One major mediator produced and secreted by endothelial cells is NO (
Figure 1). Among the numerous functional influences of NO are cardiac-related functions, including key regulators of vasodilation, reduction of permeability and thrombogenesis, and inhibition of inflammation
[25]. Another active mediator secreted by endothelial cells is CNP. Together, NO and C-type natriuretic peptide (CNP) contribute to the suppression of cardiac hypertrophy by up-regulating cyclic GMP (cGMP)-dependent protein kinase 1 (PKGI) signaling
[26] by inhibiting calcineurin (
Figure 2).
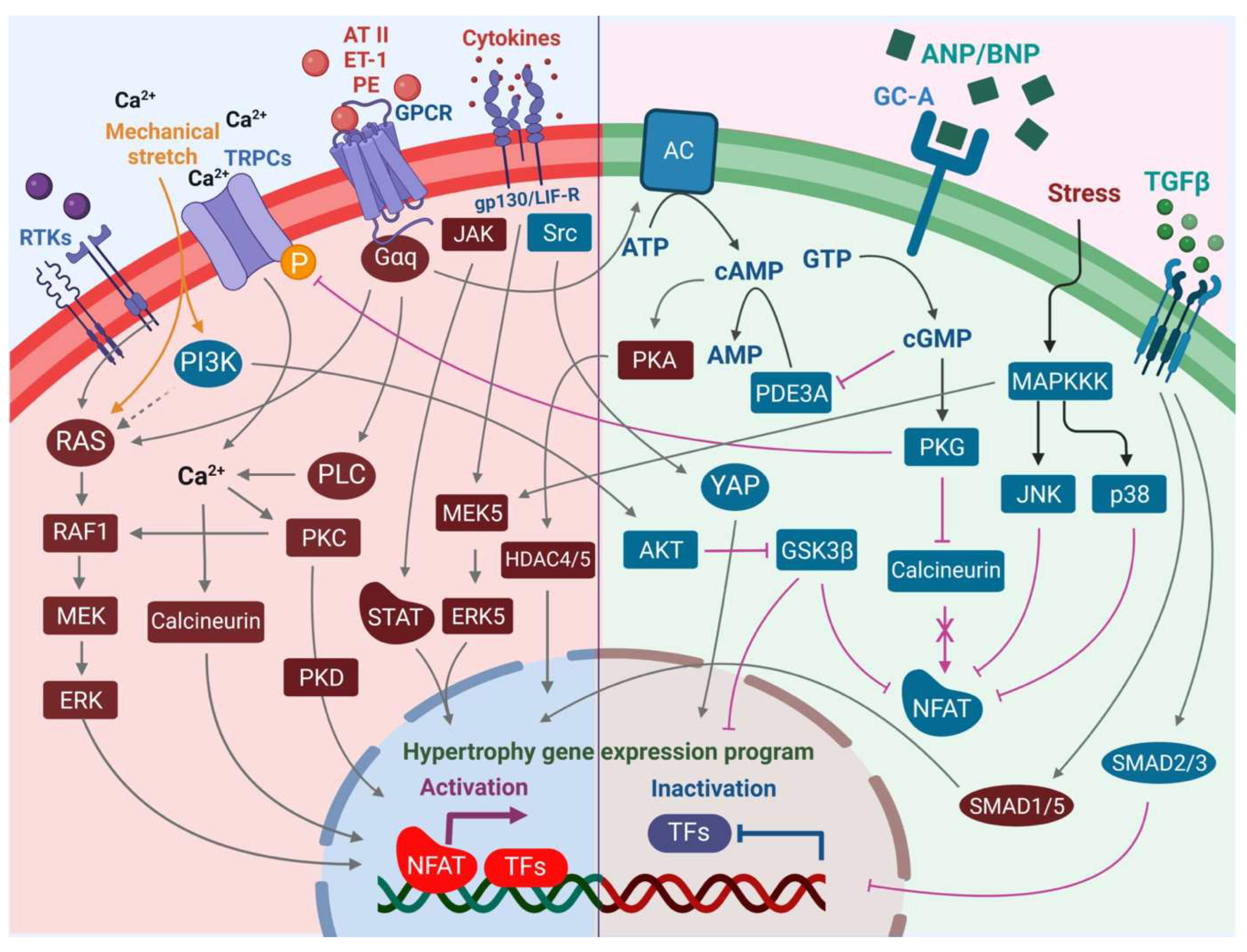
Figure 2. An overview of the pro-hypertrophic (left panel) and anti-hypertrophic (right panel) signaling pathways regulating the hypertrophic response in the cardiomyocyte. Increased intracellular Ca2+ levels mediated by TRPCs and Ca2+ import promote pro-hypertrophic transcriptional signaling events via calcineurin-NFAT and activation of PKC. PLC may contribute to these axes in the activation of alpha-adrenergic receptor signaling. Although canonical MAPK signaling via RTKs including FGFR-1 promotes pro-hypertrophic signaling, the PI3K-AKT axis plays an opposing role in hypertrophic signaling via inhibition of GSK3β and activation of YAP transcriptional activity. Increased secretion of cytokines promotes transcriptional activation of the pro-hypertrophic gene program in the nucleus not only via JAK-STAT but also the MEK5-ERK5 axis. On the other hand, increased pressure overload in cardiac tissue promotes secretion of ANP and BNP by cardiomyocytes, leading to vasodilation and an anti-hypertrophic response in cells via an increase in intracellular cGMP levels, which leads to activation of PKG, in turn mediating reduced hypertrophic growth. Activation of JNK and p38 stress signaling events in the cardiomyocytes, although leading to cardiomyopathy and heart failure, results in inhibition of NFAT through phosphorylation events that prevent its nuclear localization and pro-hypertrophic transcriptional regulation activities, thereby blocking the calcineurin axis. Increased secretion of TGF-β during increased pressure stress can lead to mixed responses, with canonical TGF-β-SMAD2/SMAD3 signaling leading to anti-hypertrophic responses, whereas activation of non-canonical SMAD1/SMAD5 leads to pro-hypertrophic responses.
Another endothelium-derived factor next to NO and CNP is ET-1
[27], which contributes to cardiac hypertrophy and fibrosis as a major growth factor. Aside from endothelial cells, ET-1 is, amongst others, also expressed in non-endothelial cells, such as fibroblasts and cardiomyocytes (
Figure 1). Functioning in an autocrine and paracrine manner, ET-1 seems to have important effects during the development of cardiac hypertrophy
[28]. ET-1 exhibits a positive inotropic effect as well as triggers cardiac hypertrophy responses
[29]. Moreover, cardiac endothelial cells carry enzymes with protease activities, like the angiotensin-converting enzyme (ACE) and chymase (
Figure 1), which may contribute to changes in local levels of AT-II
[30]. Besides fibroblasts, endothelial cells may also contribute to cardiac fibrosis (
Figure 3). For example, it is known that endothelial cells and pericytes as the capillary lining cells wrapped around them, control cardiac fibroblast numbers
[21]. Whether this contribution is similarly relevant as the proliferation and activation of resident fibroblasts upon exposure to pressure overload await further investigation
[16].
Figure 3. Schematic illustration of the process of fibrotic scar formation at the cellular level. The myocardium develops cardiomyocyte hypertrophy under pressure overload conditions, triggering concomitant inflammatory processes and fibrotic scar formation. The evidence discussed in the text suggests a central role for resident fibroblasts, nonetheless cardiac endothelial cells may also contribute to myofibroblast-like cells and drive cardiac fibrosis. Resident and infiltrating immune cells, including mast cells, macrophages, and neutrophils, enhance this phenotype change by releasing TGFß while mediating tissue inflammation via cytokines such as TNFα, IL-6, and IL-1. These mechanisms increase the number of myofibroblasts and the accumulation of collagen, which accelerates fibrotic scar formation in the microenvironment of cardiac hypertrophy.
3. The Role of Immune Cells in Cardiac Hypertrophy
3.1. Cardiac Mast Cells
Identification of the presence of mast cells in the heart tissue of animals and humans
[31][32], as well as the discovery of mast cells as the source of an array of mediators
[33], clearly emphasize the crucial participation of innate immune cells, especially cardiac mast cells, in cardiac hypertrophy and remodeling (reviewed in
[34][35]).
Activated cardiac mast cells were identified in spontaneously hypertensive rats as a major source of growth factors (
Figure 1), such as TGF-ß and bFGF, in areas of myocardial fibrosis
[36]. This is consistent with findings that the release of TGF-ß provokes an increase in collagen production alongside the differentiation of fibroblasts to myofibroblasts (
Figure 1)
[37], and indicates that cardiac mast cells also contribute to the key steps of cardiac tissue fibrosis
[38]. Another major mediator that is released upon mast cell degranulation in the heart is histamine
[31]. Histamine is a neurohormonal mediator that binds to histamine H1, H2, and H3 receptors, thereby inducing various cellular functions
[39][40] as well as cardiac hypertrophy (
Figure 1)
[41].
Another characteristic of mast cells involves their strategic location often at a perivascular site, thereby exerting regulatory functions on endothelial cells. Mast cells synthesize several endothelial cell activators comprising, amongst others, the platelet-activating factor (PAF), IL-1ß, IL-4, and tumor necrosis factors alpha (TNFα)
[42][43][44].
3.2. Monocytes & Macrophages
Healthy and injured cardiac tissues possess heterogeneous populations of macrophages, in both humans and mice (
Figure 1)
[45]. Most macrophages within the heart are established embryonically from the yolk sac and fetal liver progenitors, similar to tissue macrophages of the liver or brain. Local proliferation in contrast to monocyte recruitment serves to maintain resident macrophage subsets
[46][47]. In the absence of disease, self-renewal serves to maintain local tissue macrophage populations
[48]. Despite this, in response to pressure overload or ischemic injuries, the majority of macrophages are derived from the recruitment and differentiation of blood monocytes
[49].
Cardiac macrophages are key effector cells mediating tissue remodeling and fibrosis (
Figure 3)
[50]. The initial and significant event for vascular lesion formation results from inflammatory cytokine- and growth factor-producing migrating macrophages (
Figure 1)
[51]. The accumulation of macrophages has been found in the perivascular space, where they co-localize with fibroblasts collectively producing collagen during cardiac hypertrophy (
Figure 3)
[52][53].
3.3. Neutrophils
Under normal reparative conditions, neutrophil granulocytes are recruited to areas of acute inflammation, where they perform functions such as the clearance of dead cells and matrix debris (
Figure 3)
[54][55]. As key components of the inflammatory response, neutrophils also act on the recruitment, activation, and programming of antigen-presenting cells (APCs). Specifically, they attract monocytes and dendritic cells (DCs) by generating chemotactic signals, thereby influencing the differentiation of macrophages into a predominantly pro- or anti-inflammatory state
[56][57][58]. Because neutrophil granulocytes are one of the most important cellular components of the body for the destruction of microorganisms, there is also the possibility that these cells damage host cells and tissues
[59].
Neutrophils have been described to produce cytokines such as TNFα that drive macrophage and dendritic cell differentiation
[56][58][60]. Additionally, neutrophilic nicotinamide adenine dinucleotide phosphate (NADPH) oxidase gets activated in response to pressure overload injury
[61], resulting in the degranulation of neutrophils and thereby release of pro-fibrotic proteases (
Figure 3) as well as reactive oxygen species (ROS)
[62].
3.4. Lymphocytes
A growing body of research indicates that systemic inflammation may play a significant pathophysiologic role in the etiology of cardiac disease development, including HCM, and may have an impact on the severity of the phenotypic and clinical outcomes, including heart failure. A high neutrophil-to-lymphocyte ratio (NLR), a marker of oxidative stress damage, has been linked to an increased 5-year risk of sudden cardiac death associated with HCM
[63][64], which supported further the prognostic significance of inflammation.
However, in angiotensin II-induced HF models, the absence of B cells led to less hypertrophy and collagen deposition, the preservation of left ventricular function, and, in conjunction with these changes, a decrease in the expression of proinflammatory cytokines and apoptosis in the myocardium
[65]. Different studies have also reported that activation of NK T cells improved cardiac remodeling events and failure in mice by increasing the expression of cardioprotective cytokines, including IL-10
[66][67].
3.5. Sympathetic Neurons
Sympathetic neurons that innervate the heart and release norepinephrine (NE) also express the endothelin receptor A (ET-A)
[68][69]. ET-resulted in a tremendous NE release in cocultured cardiomyocytes and sympathetic neurons with exaggerated hypertrophy of cardiomyocytes compared to monocultured cardiomyocytes. In contrast, mice lacking the ET-A receptor exclusively in sympathetic neurons showed less adverse structural remodeling, and cardiac dysfunction when exposed to pathological pressure overload
[70].
Substantial amounts of renin released in the cardiac microenvironment upon cardiac mast cell degranulation
[71] result in both AT-II formation within striking distance of AT1 receptor-expressing cardiac sympathetic nerve terminals and enhanced NE release (
Figure 1) and arrhythmias (
Figure 4)
[72][73].
Figure 4. Schematic representation of the effects of changes in the microenvironment on cardiac function. Hypertension, a common cardiovascular disease, causes pressure overload followed by a massive release of pro-hypertrophic, pro-fibrotic, and pro-inflammatory mediators. At this stage, when individuals do not experience symptoms, hypertension, and its accompanying microenvironmental complications may be reversible with strategies such as lifestyle modification, however without any intervention, this could evolve into cardiac hypertrophy and fibrotic remodeling. Increasing fibrosis leads to mechanical stiffness and impaired filling phase, both prominent features of diastolic dysfunction. Common symptoms include headache, dizziness, palpitations, and chest discomfort. Notably, this phase is not reversible and requires pharmacological management. Late diagnosis or inadequate treatment leads to progressive fibrosis and detrimental changes at the molecular level, such as a barrier between cardiomyocytes at the cellular level, impaired electrical coupling, and hypoxia of affected cardiomyocytes, collectively resulting in cardiomyocytes’ cell death. The subsequent decreased contractile force characterizes systolic dysfunction while having severe consequences as individuals suffer from shortness of breath. Biomarker identification in a diagnostic screening approach could help detect early onset diastolic dysfunction in affected individuals, setting the platform for early management and preventive course of action to avoid the subsequent detrimental outcomes of the developing condition.
4. Mediators of Cardiac Remodeling
4.1. Activation of the Local Renin-Angiotensin System (RAS)
In addition to the classical circulating renin-angiotensin system (RAS)
[74], the heart has a local RAS that mediates autocrine, paracrine, and intracrine effects (
Figure 1)
[75][76]. Components of the RAS, including angiotensinogen (AGT), renin, ACE, AT-I, and AT-II, are expressed in the heart
[77][78], and component expression is upregulated in cardiomyocytes in vitro in response to stretch
[79][80].
4.2. Reactive Oxygen Species (ROS)
Reactive oxygen species (ROS) such as superoxide anion (O
−2), hydroxyl (OH), and hydrogen peroxide (H
2O
2), and reactive nitrogen species including nitric oxide (NO) and peroxynitrite (ONOO
−) classify reactive species involved in redox signaling. The latter results from the reaction of (O
−2) with NO
[81]. Data suggest that both direct and indirect mechanisms resulting from redox signaling within and between endothelial cells and cardiomyocytes are responsible for functional communication between these cells
[10].
In cardiac cells, several sources of ROS have been described, such as mitochondria
[82], xanthine oxidase (XO)
[83], uncoupled NO synthases (NOS)
[84], and NADPH oxidases (NOXs)
[85]. The interactions of NOX proteins with NOS-derived NO have been highlighted to be particularly important for redox signaling in the development of heart failure (
Figure 1)
[85][86][87].
An increase in the cardiac generation of ROS and therefore an increase in oxidative stress has been implicated in pressure-overload-induced left ventricular cardiac hypertrophy (LVH) and heart failure (
Figure 1)
[88][89]. Additionally, the development of cellular hypertrophy and remodeling has been found to implicate increased ROS production, and activation of the mitogen-activated protein kinase (MAPK) superfamily, where redox-sensitive protein kinases, are known to be partly responsible. Moreover, cardiomyocyte apoptosis and necrosis may be due to increased oxidative stress (
Figure 4), which is described to be associated with the transition from compensated pressure-overload-induced hypertrophy to heart failure.
4.3. Endogenous Storage Pools of AT-II in Secretory Granules
AT-II secretion into the culture medium upon mechanical stress of isolated cardiomyocytes has been observed and provides some evidence supporting the concept of increased local concentrations of AT-II
[79]. Potential autocrine and paracrine regulatory mechanisms of AT-II may activate the AT1 receptor on cardiomyocytes and surrounding cells
[90][91]. This in turn has been proposed to induce the release of autocrine and paracrine mediators, including vasoactive peptides, growth factors, cytokines, and ECM components, such as collagen (
Figure 1)
[28][92][93][94]. Potentiated or sustained AT1 receptor activation is likely associated with cardiomyocyte hypertrophy, fibroblast hyperplasia, and fibrosis (
Figure 4)
[95][96][97].
4.4. The Two Faces of the TGF-ß Signaling
AT-II-activated fibroblasts release TGF-ß and ET-1 in a paracrine manner into cardiomyocytes, leading to hypertrophy
[28]. Similar to mechanical stress, autocrine TGF-ß signaling promotes fibroblast proliferation and ECM production (
Figure 1), especially collagen and fibronectin, whereas degradation of these components is reduced
[98]. Several studies report that the canonical TGF-ß/SMAD2/3 signaling pathways (
Figure 2) induce the expression of genes related to collagen, fibronectin, and other ECM proteins
[99][100][101][102], which concomitantly contribute to cardiac fibrosis (
Figure 1)
[103]. Experiments using pressure-overload rats demonstrated that a TGF-ß neutralizing antibody inhibited fibroblast activation and proliferation, and diastolic dysfunction
[103]. These data suggest TGF-ß as a central target and the inhibition of TGF-ß signaling as beneficial. In line with this, cardiac fibrosis was attenuated in SMAD3 deficient mice subjected to cardiac pressure overload, but interestingly cardiac hypertrophy and cardiac dysfunction were aggravated
[104].
4.5. Endothelin-1 Effects
Endothelin-1 (ET-1) is an endothelium-derived vasoconstrictor of 21 amino acids. Later, two additional homologs (ET-2 and ET-3) were identified. ET-1 is released from vascular endothelium and other cells including cardiomyocytes (
Figure 1) after cleavage from a large precursor peptide
[105]. ET-1 is the predominant endothelin in the heart and is identified as a potent hypertrophic stimulus in neonatal cardiomyocytes
[106]. ET-1 is a ligand for two GPCRs: ET-A and ET-B where 90% of the endothelin receptors on cardiomyocytes belong to the ET-A subtype (
Figure 2)
[107]. In rat hearts, the ET-A is predominant and identified to be coupled to both the Gq and Gi subfamily of G-proteins (
Figure 2)
[108][109].
4.6. FGF-2 Effects in Scar Formation
In general, considering the epigenetic state and very low proliferative potential of adult cardiomyocytes, consensus exists that there is only a small ability to regenerate injured myocardium through the proliferation of cardiomyocytes
[110][111]. Instead, scar formation occurs through infiltrating highly proliferative cardiac fibroblasts (
Figure 1 and
Figure 3)
[112]. A key player is FGF-2 (bFGF), which is expressed by numerous cell types in the adult myocardium. FGF-2 is released upon cardiac injury from its “storage site” thereby potentially activating cell surface receptors, such as FGFR (
Figure 2)
[113]. Moreover, AT-II, ET-1, and FGF-2 itself are known to promote FGF-2 gene expression
[114][115].
FGF-2 null mice had a marked reduction of the hypertrophic response in cardiomyocytes in response to pressure overload
[116]; however, questions remain whether the entire blockade of FGF-2 signaling is therapeutically beneficial. Considering data highlighting the role of FGF-2 in the progression of many cancer types
[117][118][119][120][121], blocking of FGF-2 may have beneficial effects as shown in reports on the elimination of tumor angiogenesis
[122]. But, in the context of ischemic heart disease, inhibition of FGF-2 signaling may be detrimental, since an angiogenic effect by Lo-FGF-2 upregulation may be desirable
[114][123][124].
4.7. Cytokines and Inflammasome in Cardiac Remodeling
Cytokines of the interleukin-6 (IL-6) family are key molecules for the local regulation of hypertrophic responses in cardiomyocytes (
Figure 1). Pressure overload acts as a strong trigger for the upregulation of genes related to leukemia inhibitory factor (LIF) and cardiotrophin-1 (CT-1) in the adult human myocardium
[125][126]. Cardiomyocytes and cardiac fibroblasts produce leukemia LIF and CT-1
[127]. The release of Hi-FGF-2 from cardiac fibroblasts has been suggested to act in an autocrine way and trigger the release of pro-hypertrophic CT-1
[93][128]. Moreover, cardiomyocytes also express autocrine-acting CT-1, and CT-1 induces hypertrophy of cardiomyocytes in vitro
[129]. Increased production and release of LIF, CT-1, and IL-6 in cardiac fibroblasts in response to AT-II can contribute to cardiomyocyte hypertrophy by paracrine activation of the gp130-linked downstream signaling (
Figure 2)
[130].
4.8. Calcineurin/NFAT in Cardiac Hypertrophy
Calcineurin as a Ca
2+-dependent serine/threonine protein-phosphatase has been found to exhibit central pro-hypertrophic functions in the myocardium (
Figure 2)
[131][132]. Calcineurin contains two subunits: the 57–61-kDa catalytic subunit (CnA) and the 19-kDa regulatory subunit (CnB). Activation of this dimeric protein occurs through direct binding of the Ca
2+-saturated adaptor protein calmodulin
[133]. The mammalian heart only expresses CnAα, CnAβ, and CnB1, although there are three genes including CnAα, β γ encoding for CnA, and two genes (
CnB1 and B2) encode for CnB. Calcineurin becomes activated in response to increased Ca
2+ levels, which enables binding to transcription factors of the nuclear factor of activated T cells (NFAT) family (
Figure 2)
[133].
Pro-hypertrophic gene expression is activated upon binding, and through dephosphorylation of conserved serine residues at the N-terminus of NFAT by calcineurin, resulting in NFAT translocating into the nucleus (
Figure 2). Here, NFAT regulates the expression of cardiac genes via association with GATA4 and myocyte enhancer factor 2 (MEF2), which are also transcription factors
[134][135].
GPCR stimulation with hypertrophic agonists, including AT-II and PE on cultured neonatal rat cardiomyocytes indicated an increase in calcineurin enzymatic activity, which was induced by increased calcineurin Aß (CnAβ) mRNA and protein, compared to CnAα or CnAγ
[136]. By that, human hypertrophied and failing hearts (
Figure 4) also exhibit increased calcineurin activity
[137], as well as in ventricular muscle with exposure to AT-II, ET-1, and Urotensin II in human failing heart
[138].
4.9. ANP/BNP in Cardiac Hypertrophy
Development of pathological cardiac hypertrophy is frequently linked to increased mRNA expression of atrial natriuretic peptide (ANP) and B-type natriuretic peptide (BNP), according to studies in both human and animal models
[139][140], as well as an increase in the plasma levels of ANP and BNP with the severity of heart failure. Under critical conditions, more BNP than ANP is secreted, largely in the ventricles and atria, respectively. However, as heart failure worsens, ANP is also secreted in the ventricles; for this reason, the ventricles are crucial locations for both BNP and ANP
[141]. Both ANP and BNP, as well as their more stable cleavage products, NT-proANP and NT-proBNP, respectively, are efficient biomarkers in the clinical diagnosis and management of heart failure (
Figure 4)
[142][143].
Besides the physiological effects of ANP and BNP such as vasodilation, regulation of sodium reabsorption and water balance as well as inhibition of the renin-angiotensin-aldosterone (RAA) system, collectively directed towards responding to cardiac pressure and volume dynamics and suppression of heart failure
[144][145], ANP/BNP causes the cGMP-dependent PKG to be activated (
Figure 2), which in turn prompts the Ca
2+/calmodulin-dependent endothelial nitric oxide (NO) synthase to aid in the production of more NO, which relaxes the vascular smooth muscle cells and lowers systemic blood pressure
[144][146][147].
5. Mathematical Modeling of Cardiac Remodeling
5.1. Computational Models of Cardiac Hypertrophy
Several computational models have been developed to address this, providing systems-level insight into how cardiac hypertrophy is regulated. In the first model of hypertrophic signaling, Cooling et al. examined the factors that control the kinetics of IP3
[148]. They found that ET-1 induced a much more sustained IP3 signal than AT-II, which was best explained by differences in receptor kinetics. To obtain a more global view of hypertrophic signaling, Ryall et al. used a logic-based modeling framework
[149] to simulate 193 reactions integrated across 14 pathways
[150]. Comprehensive knockout simulations supported the conclusion that RAS GTPase is the hub of a bow-tie control structure, which integrates signals from many receptors and stimulates hypertrophy through partially redundant MAPK pathways. This was validated in new experiments comparing the effects of inhibition of RAS GTPase, MEK, p38, and JNK
[150].
5.2. Computational Modeling of Fibrosis
As illustrated in
Figure 2, the complexity of intracellular networks often prohibits the identification of the signaling mechanisms that control cellular responses to biochemical or mechanical stimuli upon hypertrophy. To address this challenge, Zeigler et al. developed a logic-based differential equation model of the cardiac fibroblast signaling network, which was successfully validated against 80% of 41 papers from the literature not used in model development
[151]. This model predicted that stretch-mediated myofibroblast activation was mediated not by any single path from integrins to α-SMA expression, but by an autocrine TGF-β autocrine loop. They validated this new prediction in new experiments by using a TGF-β receptor inhibitor to block cardiac myofibroblast activation in mechanically-restrained collagen gels
[151]. This model was later extended to predict the in vivo fibroblast dynamics after myocardial infarction, predicting how IL-1 can paradoxically enhance collagen production through the above autocrine TGF-β loop but suppress it through activation of NFkB and BAMBI
[152].
To predict therapeutic approaches, the fibroblast network model was integrated with DrugBank to predict FDA-approved drugs that could be repurposed against cardiac fibrosis
[153]. Interestingly, the combination drug Entresto (valsartan/sacubitril) was predicted to be particularly effective due to combined suppression of ERK through valsartan and enhancement of PKG through sacubitril
[153]. This prediction was validated by independent studies showing that Entresto decreases fibrosis due to pressure overload in rats
[154][155] and heart failure in humans
[156].