Parkinson’s disease (PD) is an age-related chronic, progressive, multi-system, neurodegenerative disease with an incidence second only to Alzheimer’s disease. A PD diagnosis requires the presence of two core motor features, including diminished movement (bradykinesia), tremor, muscle rigidity, or postural instability, difficulty initiating voluntary movement (akinesia), involuntary eye movements, and blinking, which can take up to 15-20 years to become evident. There is now an increasing level of evidence linking mitochondrial dysfunction, including elevated oxidative stress, reactive oxygen species (ROS) and impaired cellular energy production, with the overactivation and escalation of a microglial mediated proinflammatory immune response, as naturally occurring and damaging interlinked bidirectional and self-perpetuating cycles that share common pathological processes in aging and PD. This research proposes that both chronic inflammation, microglial activation and neuronal mitochondrial impairment should be considered as concurrently influencing each other along a continuum, rather than as separate and isolated linear metabolic events that affect specific aspects of neural processing and brain function.
1. Age as the Key Risk Factor of PD
While age is the key risk factor for PD
[1][2][3][4], neurodegenerative progression of PD has long been associated with escalating neuroinflammation from chronic immunological activation of the microglial system in the brain
[5][6][7][8][9], but seldom considered in terms of the concurrent effects of ageing on neurons for which substantial evidence now exists. Indeed, reduced mitochondrial membrane integrity and degradation of the energetic function of neuronal mitochondria are also inevitably linked with ageing
[4][10][11][12][13][14] and associated with innate immune system responses, which include damage-induced microglial activation
[15][16][17][18][19][20][21][22][23], signalling interactions with the vascular system
[24], and self-perpetuating feedback loops that further exacerbate neuroinflammation and escalate neurodegenerative damage in PD
[18][25]. See
Figure 1.
Figure 1. Self-perpetuating bi-directional neurotoxic feedback loops between mitochondrial impairment and microglial over-activation. Bi-directional communications between neuronal mitochondria and microglia are part of the cellular innate immune response and are implicated in central neural inflammation and the loss of dopaminergic neurons, leading to neurodegenerative progression first in the locus coeruleus (LC) and substantial nigra pars compacts (SNpC) of Parkinson’s disease patients. Mitochondrial impairment as a result of electron transport chain dysfunction, lower adenosine triphosphate (ATP) output, elevated levels of ROS, oxidative stress, increased mitochondrial membrane permeability, failure of membrane structure, and failure of phagocytotic clearance each contribute to increased cellular toxicity and apoptosis. Failure of membrane structure and phagocytotic clearance then triggers a microglial-mediated immune response to mitigate escalating inflammation because of increasing cytotoxicity. As the neuron attempts to manage increasing inflammatory damage and cytokine release, it also results in further neuronal mitochondrial impairment, triggering rising microglial activation to chronic levels, perpetuating the neuronal damage, cognitive impairment, and motor dysfunction
[26][27].
2. Inflammation and Inflammaging in Ageing
Ageing and age-related diseases such as PD share a number of basic mechanistic pillars that converge on inflammation after the age of 40
[28]. During ageing, there is overwhelming epidemiological, biological, and metabolic evidence of age-acquired immuno-dysfunction and increasing microglial density across different areas of the brain over the lifespan
[29][30][31]. Additionally, these changes are combined with an ongoing and escalating chronic, low-grade inflammation, called inflammaging
[26][32], taking place without evidence of primary infection
[33]. Beyond 40 years of age
[28], immunosenescence is responsible for the gradual and consistent rise in cumulative low-level inflammation
[34][35], with a commensurate deterioration of the innate immune system’s capacity to respond efficiently to pathogenic removal
[34], downregulation in the autophagic expression associated with compromised phagocytosis
[1], and the management and control of cellular homeostasis
[36]. The decreased activity in phagocytosis during ageing
[37] further compromises the microglia’s ability to effectively clear toxic substances from the cell and restore homeostasis
[38]. Impaired phagocytosis then leads to increased neurotoxicity and cellular senescence in response to cellular damage and stress, increasing oxidative stress and ROS, and increasing cellular death
[39][40][41][42].
Impaired activation of the adult immune system during ageing has also been linked to the pathogenesis of obesity-related insulin resistance
[43][44], type 2 diabetes
[45][46] and increased risk of autoimmune disorders, such as rheumatoid arthritis
[2][47], and vascular conditions, including hypertension (high BP) and hypotension (low BP)
[48]. Such chronic conditions are also linked to age-driven inflammaging, with its increased risk of micro and macrovascular complications, such as persistent blood pressure anomalies and small blood vessel disorders, which contribute to age-related neurodegenerative disorders such as PD
[35]. Interestingly, such age related chronic medical conditions have also been reported to show evidence of mitochondrial disease
[49][50][51][52][53][54][55][56][57], including inadequate ATP availability, dysfunction in regulating cytoplasmic and mitochondrial calcium levels, and mitophagy linked to rising toxic levels of ROS
[58]. Thus, it is no surprise that medical comorbidities such as orthostatic hypertension/hypertension, olfactory loss, sleep disruption, persistent constipation, and diabetes are highly correlated in predicting PD and the progression of the disease
[45][59][60]. See
Figure 2.
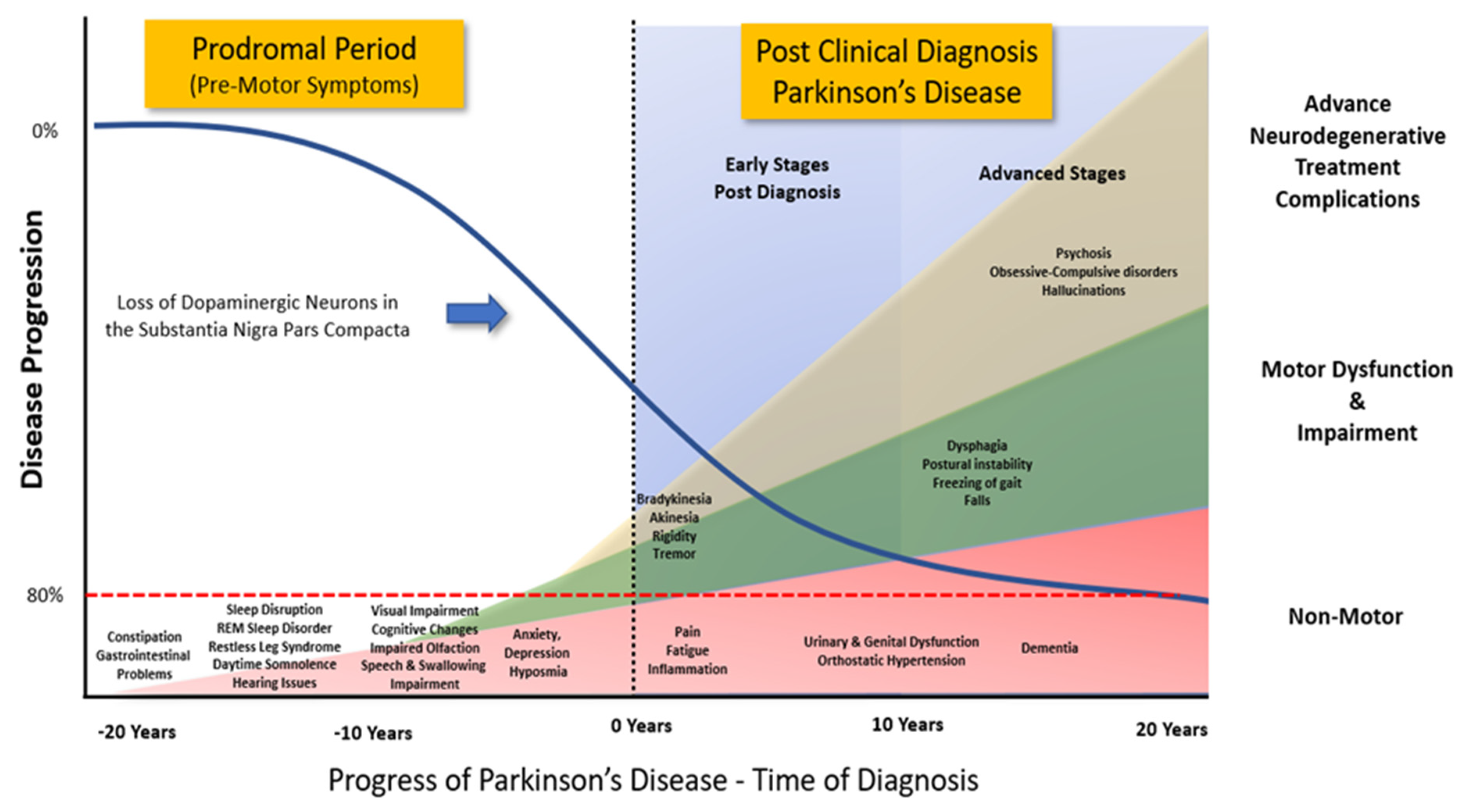
Figure 2. Progression of Clinical Symptoms from Early Prodromal through to Clinical Diagnosis of Parkinson’s Disease. Clinical diagnosis of Parkinson’s Disease accepts that there is a potential loss of up to 80% of the dopaminergic neurons in the Substantia Nigra Pars Compacta. However, prior to the emergence of any significant motor impairment, a wide variety of symptoms associated with non-motor dysfunction and disability usually precede the clinical diagnosis of Parkinson’s disease by 10-20 years. (Adapted from data and figures in Kalia et al., [61], Parkinson's disease. The Lancet, 386(9996), 896-912, and Tansey et al., [62], Inflammation and immune dysfunction in Parkinson disease.
3. Mitochondrial Dysfunction and PD
Impaired or damaged neurons from post-mortem brain samples taken from the Substantia Nigra pars Compacta (SNpC) of PD patients, show mitochondrial dysfunction in terms of increased mutations in mitochondrial DNA (mtDNA), and mitochondrial ribonucleic acid (mtRNA), loss of electron transport chain (ETC) efficiency, deficiencies in
Adenosine triphosphate (ATP) production
[63][64][65], increased proton leakage
[66][67], and increasing production of neurotoxic free radicals, such as intracellular nitrous oxide synthase (iNOS)
[68], and reactive oxygen species (ROS)
[69][70][71][72][73][74][75]. As mitochondrial integrity fails there is also an increased release of ATP and mitochondrial debris into the cytosol
[76][77], See
Figure 3. As these molecules are released into the cytosol they interact with the same specialised cytoplasmic sensors that detect cellular pathogens, triggering a microglial-mediated inflammatory immune response
[78] within the SNpC, leading to apoptosis and the loss of dopaminergic neurons. The release of mtDNA, mtRNA, and ATP into the cytosol also activates various immune signalling receptors, including Toll Like Receptor (TLR9), Nod Like Receptor (NLRP3), and Stimulator of interferon genes (STING), which are involved in facilitating and regulating antibacterial and antiviral immunity as part of the proinflammatory immune response
[79]. In addition, the loss of cellular energetics has been linked to lower immune system efficiency, resulting in reductions in natural killer (NK) cells, total CD8+ T cells, and CD8+ memory T cells associated with evidence of higher viral and bacterial infection being revealed in patient case histories
[11][80]. Similar results have been reported when using immortalised lymphocytes isolated from idiopathic PD patients
[81][82], where mitochondrial mass, genome copy number, and membrane potential in the lymphoblasts were found to be functionally normal but hyperactive and producing significantly elevated levels of neurotoxic damaging ROS in PD patients compared to healthy controls.
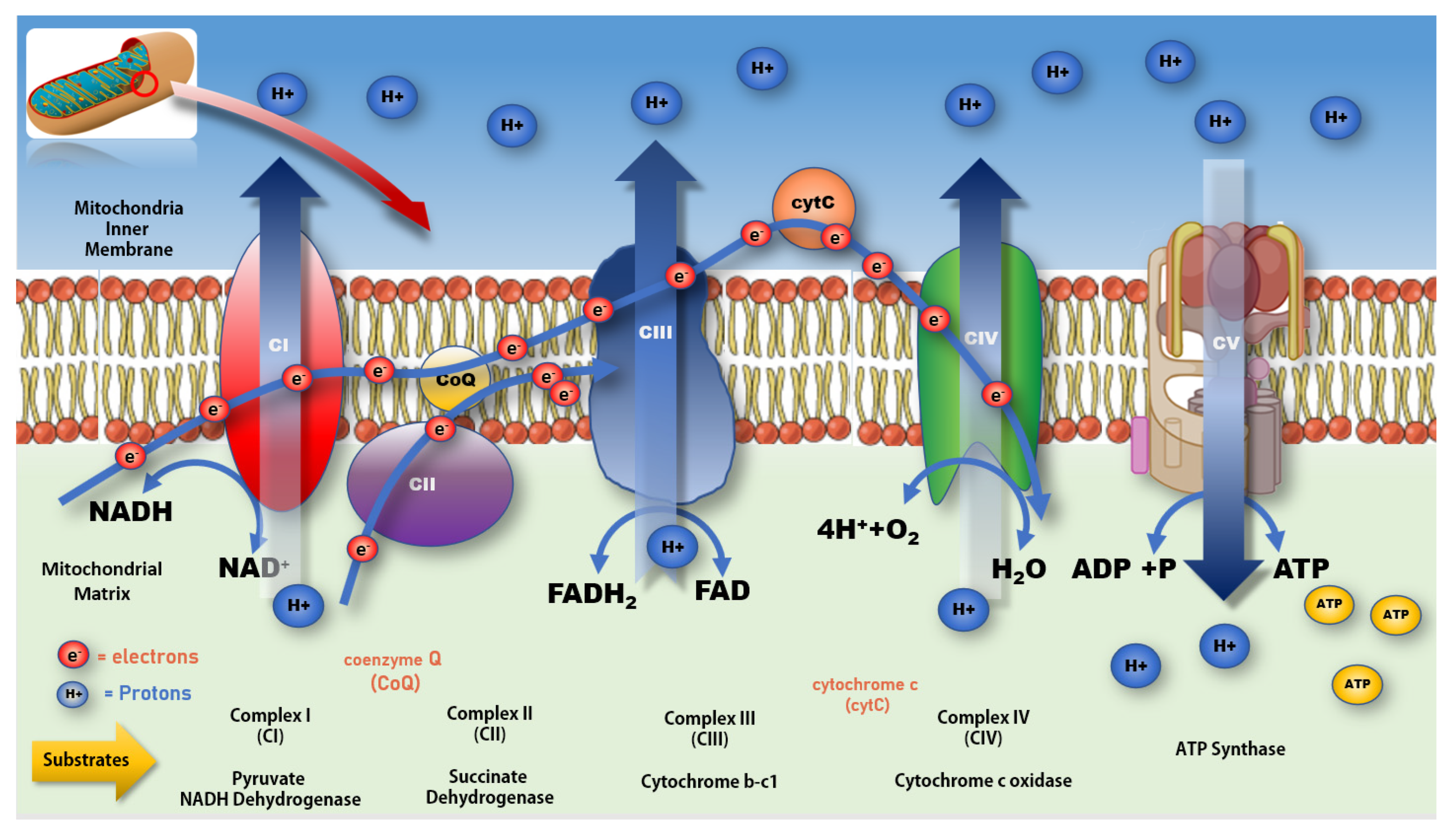
Figure 3. Mitochondrial electron transport chain and production of ATP. Impeding the flow of electrons along the ETC at CI increases production of free radicals resulting in a rise in oxidative stress and lower production of ATP at CV. A more complete review of mitochondrial bioenergetic function and associated impacts on various disease processes can be found in Protasoni et al. 2021 [70].
Dopaminergic axons in the SNpC have one of the largest energetic demands in the body; however, they also have limited surplus or reserve energetic capacity
[83]. In order to maintain efficient basal metabolic functioning
[84][85], synaptic transmissions, and cell survival
[86], dopaminergic neurons require consistent and regular supplies of mitochondria and ATP, which are met by the neuron maintaining high concentrations of mitochondria at the axon terminal. Any interruption or insufficient energetic availability can be catastrophic for the cell, resulting in significant cellular damage, apoptosis, and progressive neuronal degeneration in PD
[87]. In mature neurons and presynaptic structures, around 87% of mitochondria are stationary, residing in a highly structured reticular state
[88], and need to be transported along axons in the neuron to areas of high energetic demand. Any disruption to the movement of mitochondria along the axon via microtubule-based transport and anchoring substrates
[88] can be damaging to mitochondrial redistribution to manage physiological or pathological cellular stress in order to maintain energic homeostasis
[89][90]. Research findings by Ray, et al.
[91] have found disruption of mitochondrial crosstalk between organelles, including endoplasmic reticulum (ER), peroxisomes, and lysosomes, triggered significant changes in intracellular vesicle transporters and calcium buffering, which were implicated with increased protein misfolding, protease activation, and impaired autophagic clearance, all of which are key processes contributing to neurodegenerative progression in PD
[17].
Mitochondrial damage has critical implications for the maximum amount of ATP that can be produced and made available to the cell under pathogenic stress, and required to maintain cellular homeostasis and interactions with other cellular and vascular innate immune processes
[92]. Given the mitochondria’s low energy storage capacity and minimal surplus ATP availability, any interruption or deficiencies in the energy supply to the axons will impair or halt energy-driven neuronal events, leading to irreversible dopaminergic loss and neurodegeneration in the SNpC
[93][94]. Functional magnetic resonance imaging (fMRI), magnetic resonance spectroscopy (MRS), and positron emission tomography (PET) studies support these findings, showing that PD patients achieve significantly lower brain glucose utilisation, suggesting lower ATP production and availability in the brain regions most affected by energetic loss, such as the SNpC in PD progression
[95]. The lack of brain glucose uptake is also directly linked with subsequent cognitive decline in neurodegenerative diseases such as PD
[96].
The evidence presented here demonstrates strong causal associations between mitochondrial dysfunction and impaired ETC efficiency, resulting in excess production of neurotoxic ROS and inducible nitric oxide synthase (iNOS), microglial-mediated proinflammatory cytokine release, neuroinflammation, and progressive neuronal cell death both in aging and in PD patients. These relationships are continuously and dynamically changing in response to the cellular environment, and resulting in concurrent bidirectional associations in key signalling and molecular mechanisms linked to diminished ATP availability and impaired neuronal homeostasis, with direct consequences linked to activation of microglia and progressive neurodegeneration in PD. As a consequence of the fundamental multiple functional roles that mitochondria power in the cell, any impairment or dysfunction can often appear early in neurodegenerative progression, potentially offering a metabolic target to assist in the prodromal diagnosis of aging related PD
[93].