Since SIFT-MS is based on mass spectrometry (MS), it is significantly more complex than most chemosensors—as reflected in price differentials up to three orders of magnitude (in the case of the lowest cost chemosensors). All MS techniques require that analytes be ionized. In the case of SIFT-MS, very soft chemical ionization (CI) is utilized. As with other MS techniques, once ionized, the gas-phase ion products are subsequently separated by their mass-to-charge ratio (m/z) and then counted—the ion abundance being proportional to analyte concentration. The spectral “fingerprint” generated by the MS instrument is determined by the elemental composition of the analyte and the ionization energetics.
2. The SIFT-MS Technique—An Overview
2.1. Instrument Overview
All SIFT-MS instruments have three zones. In the first region, reagent ions are generated continuously in a plasma created at low pressure using a microwave discharge. For the positively charged reagent ions, H3O+, NO+, and O2+•, the ion source is operated at approximately 54 kPa (400 torr), while for the negatively charged reagent ions (O−•, O2−•, OH−, NO2−, and NO3−) it is operated at approximately twice this pressure to facilitate electron attachment. Multiple reagent ions provide two significant benefits that are elaborated below: (1) their different ionization properties enable a wide range of compounds to be detected, and (2) they are foundational to achieving specific analysis. The plasma containing a mixture of potential reagent ions is then passed into a quadrupole mass filter (QMF) that, using software control, selects the appropriate, single-reagent ion type (by its m/z; e.g., H3O+ with m/z = 19) for introduction as a pure stream of reagent ion into the second region, the reaction chamber (or flow tube).
In the flow tube, reagent ions first encounter carrier gas molecules (either helium or nitrogen, depending on instrument application). Through collisions with the carrier gas, the energies of the reagent ions are reduced to approximately the temperature of the carrier gas itself (i.e., the reagent ions are “thermalized”)
[5]. The sample is introduced after reagent ions have been in the flow tube for approximately 1 ms, then has a residence time of about 3 to 8 ms (configuration-dependent) for reaction with reagent ions. At the end of the flow tube, ions are sampled into the third region (detection), while the bulk of carrier and sample mixture is pumped away to exhaust. Note that the carrier gas and the bulk matrix must have ionization properties that render them essentially non-reactive with the reagent ions. Conveniently, this is the case for air, with which the reagent ions listed above either do not react or react only very slowly (nitrogen, oxygen, argon, carbon dioxide, and water).
The detection region comprises, firstly, an ion guide that improves transmission of heavier product ions, second a QMF that transmits just the ions with a given
m/
z, and finally a particle multiplier detector that counts ions at each
m/
z. Typically operation in the linear range means that less than 10% of the reagent ion signal is consumed
[6] and concentration is proportional to the ratio of product ion count divided by reagent ion count (Section 2.4). This is essentially an auto-normalization feature, correcting for any drift of ion signal, should it occur, and supports stable long-term operation.
SIFT-MS instruments are completely computer controlled and can operate autonomously or via remote control. The stability of ionization means that—when required—re-calibration is an infrequent task (annual for many compounds due to drift less than 10%), and software supports the generation of laboratory-grade analytical results for non-technical operators for properly developed analytical methods.
2.2. Breadth of Analysis
Although, as noted in the previous subsection, the SIFT-MS technique is ‘blind’ to the bulk components of air due to the low ionization energies of its reagent ions, it has remarkable breadth of analysis for VOCs and trace inorganic gases. This is due to the multiple reagent ions that ionize compounds via a wide range of ion–molecule reaction mechanisms (
Table 1). These mechanisms have been reviewed in detail elsewhere
[5][6]. Essentially, if the ionization properties of the target compound are a match for SIFT-MS, then it will be ionized and detected. Note, however, that unlike the electron ionization (EI) method used commonly with GC or photoionization detectors (PIDs), the different mechanisms yield a wider range of product ions, potentially increasing specificity for isobaric compounds.
Table 1. The most common ion–molecule reaction mechanisms of the SIFT-MS reagent ions and the resulting product ion m/z, either relative to the molecular weight or a standard product ion.
Table 2 illustrates the breadth of SIFT-MS analysis by giving examples of selected compounds that are practicably detectable in air using the indicated reagent ions. Assuming that specificity is achievable using SIFT-MS (see the next subsection), virtually any combination of compounds can be detected in a single analysis. This is possible with SIFT-MS due to the diversity of the ion chemistry and the broad-spectrum ion detection provided by mass spectrometry, coupled with removal of complications imposed by the use of chromatographic columns in conventional laboratory techniques. The subsequent sections describe applications where this multicomponent analysis of gas-phase compounds with diverse ionization properties is very beneficial.
Table 2. Examples of compounds and the SIFT-MS reagent ions by which they are practically analyzed (i.e., sufficient sensitivity and selectivity).
Since a substantial breadth of literature describes volatile compounds that are detectable using SIFT-MS (see, for example, very convenient review articles
[6][23][24]), several examples of important compounds that are not analyzable, or have significant constraints, are discussed here.
First, consider methane which is of significant concern for climate due to its high global warming potential
[25]. In positive ion mode, the O
2+• reagent ion reacts with methane, as shown in Equation (1), whereas H
3O
+ and NO
+ are too inefficient to be useful
[26][27]. However, the sensitivity of SIFT-MS is lower than for most volatiles due to the slow reaction rate coefficient (
k)—over 200-fold lower than typical
k for VOCs. Nevertheless, methane analysis has been demonstrated for breath
[27] and results for landfill and biogas analysis were reported to commercial customers by the present researcher over a 14-year period. Due to the low sensitivity, great care needs to be taken to ensure that the methane product ion does not suffer from interference.
O2+• + CH4 → CH2O2H+ + H• k = 5 − 5.5 × 10−12 cm3 molecule−1 s−1 (1)
Interestingly, O
−• reacts with methane somewhat more rapidly (Equation (2))
[28] via the hydrogen atom transfer mechanism (
Table 1). However, the product ion is also formed from reaction of O
−• with water
[28] and other low-molecular-weight hydrocarbons
[28], so it is not of practical use for specific detection of methane.
O−• + CH4 → OH− + CH3• k = 0.8 − 1.1 × 10−10 cm3 molecule−1 s−1 (2)
Second, carbon monoxide is toxic
[29] and exposure is of significant concern in confined spaces and in the urban environment. Unfortunately, of the standard SIFT-MS reagent ions only O
−• reacts with CO
[28] and this solely via the associative detachment mechanism (
Table 1). As shown in Equation (3), no useful ion is obtained since free electrons (e
−) are not detectable in SIFT-MS instruments.
O−• + CO → CO2 + e− k = 5.5 − 7.3 × 10−10 cm3 molecule−1 s−1 (3)
Finally, nitrous oxide (N
2O)—another important greenhouse gas
[25]—reacts with O
− as shown in Equation (4)
[28]. In principle, this reaction rate coefficient provides adequate sensitivity for trace gas analysis. However, in air the NO
− product ion reacts with O
2 to form O
2−• (Equation (5))
[28], which cannot be distinguished from O
2−• formed at trace levels from other sources. N
2O is hence detectable, but it is only practicable to do so in oxygen-free matrices (e.g., in specialty gas applications).
O−• + N2O → NO− + NO k = 1.95 − 2.5 × 10−10 cm3 molecule−1 s−1 (4)
NO− + O2 → O2−• + NO k = 5 × 10−10 cm3 molecule−1 s−1 (5)
Despite these limitations, SIFT-MS has found broad applications
[6][23][24]. Later sections will review applications that are more closely aligned with chemosensor technologies.
2.3. Specificity of Analysis
Achieving specific analysis in moderately complex matrixes can be challenging for low-cost chemosensors. Eminent flavor chemist, Gary Reineccius wrote of the application of electronic noses (sensor arrays) in food flavor analysis that “one has no clear idea of what the instrument is responding to in making a judgement”
[30].
In contrast, SIFT-MS achieves specific analysis in real-time by the combination of highly controlled, ultra-soft CI and MS, producing signals that are attributable to specific analytes.
Table 1 illustrates the variety of common reaction mechanisms that occur for the standard SIFT-MS reagent ions and indicates the relative shifts from parent product ion
m/
z, or standard product ion
m/
z, for these mechanisms. Combining this behavior with the observation that different functional groups typically react in different ways
[5][6], at least one unique ion for a given analyte is usually realized.
It is important to reemphasize that when using the phrase “real-time specificity” in the context of SIFT-MS, a user is not limited to a single reagent ion in each analysis. By means of the first QMF (
Figure 1), combined with software control, reagent ions generated in a given ion source setting (e.g., positive ions with H
3O
+, NO
+, and O
2+•) are switchable in tens of milliseconds. This means that target compounds can be analyzed specifically across different reagent ions, if necessary. This contrasts with the other DIMS techniques
[3]. Note that where an ion source setting change is required—e.g., from positive ions to either of the negative ion settings—this is still near real time at approximately 10 s.
Figure 2 summarizes the elements that contribute to specific analysis in SIFT-MS. Addition of a time-of-flight (TOF) mass spectrometer to SIFT-MS instruments (as has been done successfully for PTR-MS
[3]) could further improve specificity, but at additional cost. However, regardless of the way that mass filtering is accomplished in SIFT-MS or PTR-MS, it is not reasonable to expect that the specificity of DIMS techniques will approach that of GC/MS with its temporal separation of analytes. GC/MS techniques—especially those utilizing high-resolution mass spectrometry—will remain the technique of choice for compound identification and analysis of very complex matrices.
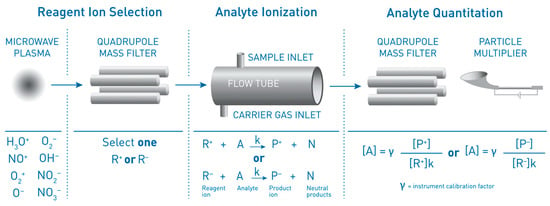
Figure 1. A schematic illustration of the SIFT-MS technique, showing the three zones in this continuous-analysis approach. Used with permission of Syft Technologies.
Figure 2. Specificity in SIFT-MS analysis is achieved through a unique combination of attributes.
Since detailed examples of SIFT-MS specificity are given in Refs.
[31][32][33][34], one simple example suffices here. Consider two structural isomers of molecular formula C
2H
6O and molar mass 58.0791 g mol
−1: acetone (propan-2-one, (CH
3)
2CO) and propanal (propionaldehyde, CH
3CH
2CHO).
Table 3 shows the primary product ions formed when acetone and propanal react with the three positively charged SIFT-MS reagent ions
[10]. Use of the NO
+ reagent ion provides the most convenient resolution of these isomers. The H
3O
+ reagent ion cannot resolve the compounds, and matrix effects are encountered frequently for the unique O
2+• product ions.
Table 3. Primary product ions formed from reaction of acetone and propanal with H
3O
+, NO
+, and O
2+• [10]. The NO
+ reagent ion is most frequently utilized to achieve selective analysis.
Table 3 ignores the so-called secondary chemistry of acetone and propanal—only the primary ions formed because of reagent ion reaction with the analytes are shown. These primary product ions can react with matrix species—most commonly water, though carbon dioxide is common for negative ions—to form secondary product ions
[5][6]. Secondary reactions are commonly association reactions (
Table 1), requiring that a third molecule (a so-called “third body”) is present in the reactive collision because it carries away sufficient kinetic energy for adduct formation to occur. The association mechanism is hence sensitive to the temperature and pressure in the SIFT-MS instrument flow tube
[5][6]. The secondary reactions pertinent to these analytes occur for the H
3O
+ product (the most common situation):
In these equations, M represents the necessary third body and the secondary product ions are located +18 units higher than the primary product ion (i.e., at m/z 77). When they occur, it is necessary to measure the signals of secondary product ions for correct quantitation (see the next subsection). Method development should also ensure that they are not interfering with other analytes nor interfered with by other analytes.
2.4. Quantitation in SIFT-MS
The preceding subsections have described how SIFT-MS can analyze a wide range of compounds and generally do so with high specificity. It remains, then, to outline how quantitative results are obtained in SIFT-MS. Since the details of the gas-phase kinetic theory have been described elsewhere
[6][35], and the detailed calculation of concentration presented
[36], only a brief sketch will be given here. A future article will describe the calculation, as currently adapted in commercial SIFT-MS instruments, in more detail. In this entry, the use of non-SI pressure units (torr) follows that of the SIFT-MS literature
[36].
The master equation used in commercial instruments to determine the concentration of analyte A in air ([A]i in units of parts-per-billion by volume, ppbV) for each primary product ion (i) of A utilized in the analytical method is:
Here:
-
TFT is the temperature in the flow tube in Kelvin (K)
-
PFT is the pressure in the flow tube in torr
-
kj is the rate coefficient for reaction of reagent ion Rj+ with the analyte (in cm3 molecule−1 s−1)
-
tr is the reaction time (in seconds)
-
φcarr is the carrier gas flow in torr L s−1
-
φsamp is the sample flow in torr L s−1
-
Pi+ is the primary product ion signal (in counts per second, cps) for primary product ion i counted by the particle multiplier detector
-
TFProd(i) is the transmission factor for the primary product ion Pi+ (dimensionless)
-
Pki+ is the secondary product ion signal (in cps) for secondary product ion k derived from primary product ion i
-
TFProd(ki) is the transmission factor for the secondary product ion Pki+ (dimensionless)
-
Rj+ is the reagent ion signal (in cps) for the injected reagent ion (j = 0) and its water cluster ions (if appropriate; j = 1, 2, 3)
-
TFReag(j) is the transmission factor for the reagent ion Rj+ (dimensionless)
-
bri is the branching ratio for primary product ion i (0 < i ≤ 1 for calculation purposes, but ordinarily tabulated as a percentage; see, e.g., Table 3).
The coefficient (1.035 × 10−10) in Equation (8) is derived from several physical constants and unit conversions and has units of Torr cm3 molecule−1 K−1. Note that the concentration [A]i is directly proportional to the ratio of measured product ion signal to measured reagent ion signal, which overcomes any ion source drift, should that occur, and confers measurement stability compared to MS detection coupled with chromatographic techniques. Clearly for correct calculation of [A]i the appropriate secondary ions need to be included in the analytical method (e.g., Equations (6) and (7)), otherwise the concentration will underreport.
The parameters in Equation (8) are obtained from various sources:
-
Instrument operating parameters: TFT, PFT, φcarr, and φsamp
-
The instrument’s automated performance check on a certified gas standard: tr, TFProd(i), TFProd(ki), and TFReag(j)
-
Software library: kj and bri (clearly, together with the m/z at which the relevant reagent and product ions will be located)
-
Measurement of sample: Pi+, Pki+, and Rj+.
When more than one concentration determination is made for analyte A (i.e., several primary product ions are used in the method), then instrument software supports automated interference rejection through comparison of the [
A]
i values (within a user-chosen tolerance; by default, 20%)
[37]. The most common failure mode for this approach is when secondary chemistry is omitted from the analytical method.
Several brief comments must be made to conclude the brief overview of SIFT-MS. First, SIFT-MS is quantifying compounds based on unique reagent ion-product ion pairs, not on pattern recognition from a sensor array—the analyst knows which compound is being detected using SIFT-MS, in contrast to sensors
[30]. This confidence is evident in the extensive SIFT-MS literature reviewed elsewhere
[5][23][24]. Second, several publications have compared SIFT-MS with gold-standard chromatography-based techniques and have reported favorable results, for example, Refs.
[38][39][40]. Third, SIFT-MS analysis can be validated according to industry standard procedures (e.g.,
[41][42]), with only minor adaptations required despite the significant change in analytical approach from chromatographic separation to DIMS. Finally, the CI approach underpinning the SIFT-MS technique provides a wide linear range
[6][43]. Ensuring that the instrument remains in the linear range is important for reliable concentration determination
[6], although calibration and related approaches can circumvent this restriction when used with care
[44]. Generally, however, SIFT-MS measurements do not drift with changes in matrix as chemosensors may. Hence SIFT-MS might provide an alternative to chromatographic methods for benchmarking chemosensors since it provides high-quality analysis in real-time.