Glycerol can be found in three different forms: commercially produced glycerol, purified/refined glycerol, and crude/waste glycerol. The purity of refined and synthesized glycerol is higher than that of crude glycerol. While synthetic glycerol is produced in a separate way, often from propene, crude and pure glycerol are byproducts of the manufacturing of biodiesel.
3. Glycerol Upgrading
Since glycerol is available as a comparatively low-cost, high-volume biomass-derived waste, the valorization of crude glycerol which is underutilized is necessary for the economic viability of biodiesel manufacturing and waste fats/oil mass production. Due to its non-toxic, edible, biodegradable, and multifunctional qualities, glycerol is one of the top building block chemicals for organic synthesis from biomass
[17]. The highly desirable molecule glycerol is utilized to make a wide range of beneficial chemical intermediates. Traditionally, one of the primary technologies for valorizing crude glycerol was through direct combustion approach. Such practice is inconvenient due to the production of acrolein, a hazardous combustion product of crude glycerol with low boiling point (53 °C) and high autoignition temperature (234 °C), and excess salt content
[18]. When compared to other feedstocks, the volatile organic compound (VOC) emissions were notably greater, albeit with swirl refractory burners. Owing to its toxic potential, the acrolein produced throughout this technique has caused a lot of environmental concern
[19]. Additionally, this technique of valorization falls short of utilizing full prospects of crude glycerol, which may be exploited through different high-end technologies.
In this regard, extensive research has been conducted over the past ten years on the chemical conversion of glycerol into specialized compounds with high added value
[20]. Additionally, because glycerol has a high degree of functionalization, it can be used as a precursor for the production of a variety of common chemicals, including syngas, alkenes, alcohols, diols, ethers, acids, esters, acrylates, and even polyglycerols by the use of a variety of processes, involving thermochemical approaches of pyrolysis, biological techniques—fermentation and microbial conversion, physicochemical methods of etherification, trans- and esterification, electro oxidation, hydrogenolysis, dehydration, carboxylation, halogenation, polymerization, and glycerol acetalization
[21], as depicted in
Figure 4. Thus, in this section, existing glycerol upgrading technologies will be summarized and compared in terms of its state-of-the-art, intended end-products, conversion efficiency, and their process condition.
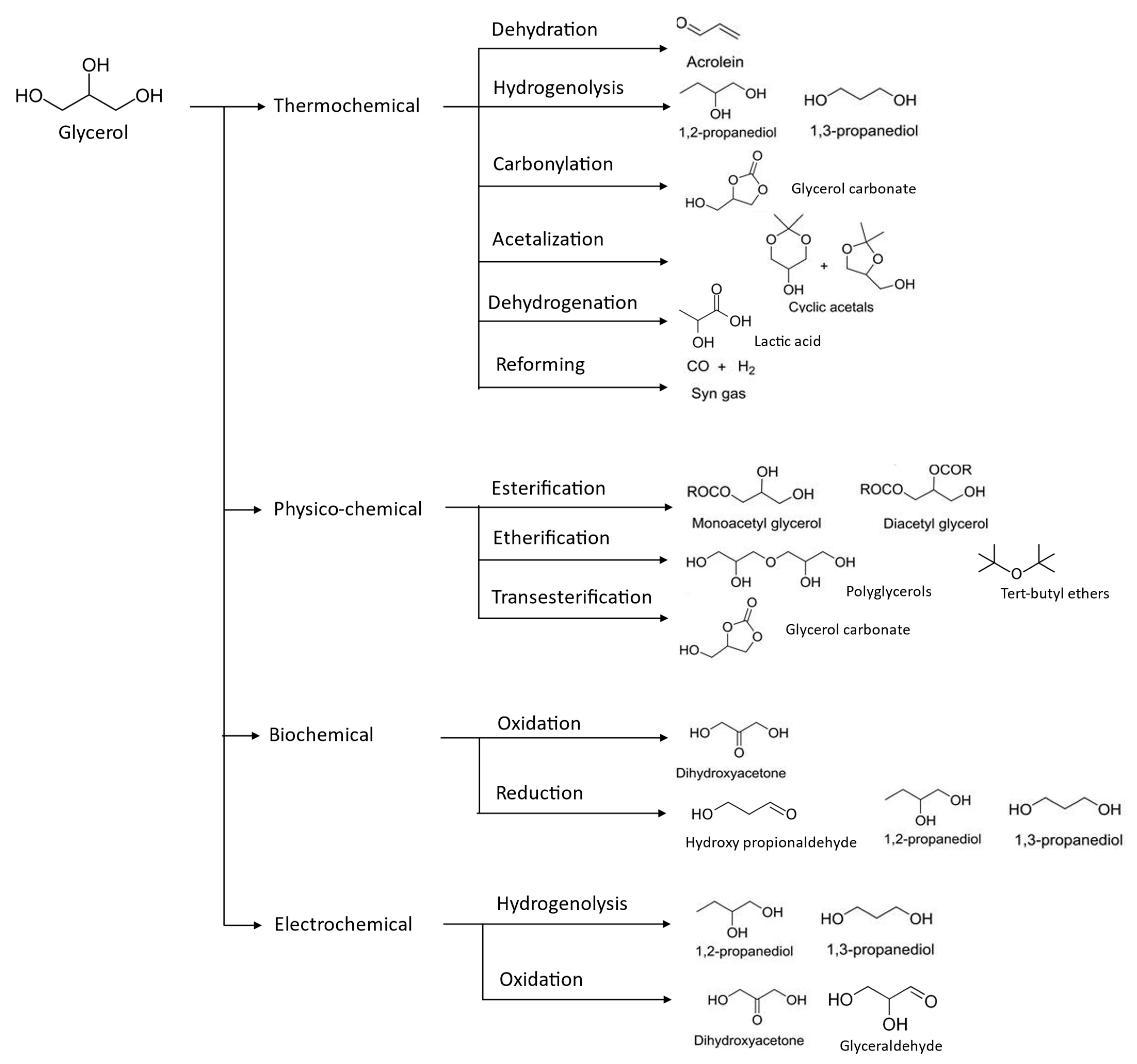
Figure 4. Valorization of glycerol compounds into various intended end-products through different chemical reaction.
3.1. Biochemical Approaches
3.1.1. Microbial Fermentation—Anaerobic and Aerobic Digestion
Crude glycerol can also be converted biologically by fermenting with various microbial biocatalysts including yeast, fungi, bacteria, mixed culture from wastewater, and microalgae under aerobic or anaerobic conditions to produce valuable chemicals. Recent studies have concentrated on using crude glycerol as a carbon source in microbial fermentation to produce green compounds and other platform chemicals
[22][23][24]. Thus, such bioconversions via biochemical approaches with the aid of microbes are a promising resource and subsequently enhances the techno economical value of the biodiesel industry. Some advantages of biological conversion via aerobic or anaerobic fermentation are due to their superior yield, selectivity, and product recovery
[25]. However, due to factors including pathogenicity, stringent anaerobic conditions, contaminants in the substrate, and lower kinetic reaction which have resulted in longer reaction times may impair their growth and conversion rates. Plus, the capacity for these organisms to be manufactured at an industry scale may also hinder their further application.
- 1.
-
Bacteria
Clostridium,
Klebsiella,
Komagataella,
Lactobacillus,
Lipomyces,
Escherichia,
Candida, and
Raoultella are a few often used glycerol-consuming species of bacteria used to manufacture valuable products either in aerobic, anaerobic, or microaerobic condition
[25]. The oxidative and reductive metabolic pathways, which also result in the formation of organic acids and alcohols, are used to assimilate glycerol
[26].
Clostridium butyricum [26][27][28][29] and
pasteurianam [30][31][32],
Klebsiella oxytoca [33][34] and
pneumonia [35][36][37][38][39],
Citrobacter freundii [40][41] and
werkmanii [42],
Lactobacillus diolivorans [43],
Enterobactor sp.
[44] as well as engineered
Escherichia coli [45][46][47] have been widely utilized in glycerol conversion into 1,2- and 1,3-PDO. From the aforementioned previous studies of various bacteria, it can be summarized that high PDO yields have been recorded, approximately 0.62–1.09 mol/mol glycerol, with inconsistent process productivity of 0.92–10.3 g/L/h
[27][28][29][30][48]. Tang et al.
[47] have fabricated an engineered
E. coli with additional genes for the production of 1,3-PDO, B12-independent glycerol dehydratase (DhaB1), and its activating factor (DhaB2) from
C. butyricum. The final PDO yield, productivity, and conversion rate recorded were 1.09 mol/mol, 2.61 g/L/h and 90.2% (g/g), respectively. Johnson and Rehmann
[31] investigated the effect of decreasing pH during fermentation of crude glycerol with
C. pasteurianum which led to the decreased in cell growth rate, CO
2 production, and slower fermentations, thus resulting in higher butanol and PDO yields in a continuous process.
For lactic acid generation, engineered
E. coli has been widely used in converting crude glycerol as an alternative to nicotinamide adenine dinucleotide (NAD
+) regeneration in the absence of external electron acceptors
[49]. The biological route of crude glycerol upgrading to lactic acid assisted by microbes stimulates the development of one specific configuration of either D- or L-, because of the excellent selectivity of lactate dehydrogenase (LDH)
[50]. Based on Mazumdar et al.
[49][51],
E. coli has been engineered to improve its efficiency towards the conversion of glycerol to D- and L-lactic acid under microaerobic and anaerobic conditions. The overall yield of 0.85 and 0.93 mol/mol glycerol has been recorded for D- and L-lactic acid, respectively, with 34 g/L and 50 g/L of each final concentration, whereas Hong et al.
[52] utilized
E. coli AC-521 to transform glycerol into lactic acid under the aerobic condition at optimized fermentation conditions of 42 °C, pH 6.5, and 0.85 min
−1 (K
LA). The overall lactic acid concentration and glycerol consumption peaked at 88 h of fermentation, resulting in 86.0 g/L lactic acid yield with 0.97 g/L/h productivity as well as a yield of 0.9 mol/mol glycerol. Other than
E. coli, there are also studies relating to the utilization of
L. casei NCIM 2125
[53],
K. phafii Glpard
[54], and yeast
S. cerevisiae [55] under fed-batch fermentation for lactic acid production.
The potential butanol producer, according to previous studies
[30][48],
C. pasteurianam under optimal condition can generate 0.43 mol/mol of butanol with 0.074 g/L/h of process productivity in 120 h using crude glycerol as a substrate. In a different study by Saini et al.
[56],
E. coli was used to assist the fermentation of crude glycerol into butanol with 0.35 mol/mol of butanol yield. Several type of bacteria were also utilized in the production of ethanol via fermentation of crude glycerol which includes
E. coli SS1
[57],
E. aerogenes TISTR 1468
[5],
Pachysolen tannophilus [58], and engineered
K. pneumonia [59] where they resulted in 1.00, 0.59, 0.56, and 0.89 mol/mol product yields, respectively. In addition, there is also a study of glycerol upgrading aided by
Saccharomyces cerevisiae yeast
[60] with an overall yield of 2.4 g/L ethanol recorded which proves the possibility of yeast in ethanol production.
- 2.
-
Microbial mixed cultures and other bacteria
Mixed culture communities have also been studied for their potential to upgrade crude glycerol into other value-added products which include hydrogen gas and PHAs. According to the literature review, the production of H
2 was dominantly covered by fermentation of mixed culture extracted from wastewater or resulted from the mixing of rare strain types. Theoretically, crude glycerol fermentation provides a better capacity to generate hydrogen gas at the end of its reaction as compared to glucose fermentation due to it generating more NADH on a 3-carbon basis where one mole of hydrogen gas per mole of excess NADH. According to previous work, Mabutyana and Pott
[61] extensively studied the homofermentative H
2 production via co-fermentation of glycerol with phenolic compounds assisted by
Rhodopseudomonas palustris under anaerobic conditions at temperature of 35 °C equipped with tungsten light bulbs. According to Varrone et al.
[62] and Chen et al.
[63], the application of enriched activity sludge or microbial mixed culture efficiently enhanced the conversion of crude glycerol into H
2 with an approximate yield of 0.52–0.96 mol/mol glycerol, whereas
Paenibacillus macerans [64] and
Thermoanaerobacterium sp.
[8], in another study, were also utilized in the fermentation of crude glycerol to produce H
2 which resulted in 0.81 and 0.30 mol/mol of yield. In addition, crude glycerol may be used as a substrate for anaerobic digestion to produce biogas. In the acidogenesis and acetogenesis phases, it is degraded to volatile fatty acids (VFAs), and in the final methanogenesis stage, methane is produced from either acetic acid, CO
2, or H
2 by the methanogenic community
[65]. For further development, anaerobic process optimization to produce hydrogen from crude glycerol is very essential and needs to be explored thoroughly.
- 3.
-
Fungi
Fungi are another promising biocatalyst to enhance the microbial conversion of crude glycerol into other specific valuable products such as single-cell oil (SCO). According to Chatzifragkou et al.
[27], throughout the fermentation process, fungi tend to build up lipids inside their mycelia. Andre et al.
[24] studied the yield of SCO and oxalic acid from the fermentation of crude glycerol with
Lentinula edodes strains and
Aspergillus niger strains in carbon-limited and nitrogen-limited conditions, respectively. The maximum yield of lipid of 3.5 g/L and 0.1 g/g biomass was recorded. The SCO product was dominantly composed of oleic acids.
Galactomyces geotrichum and
ascomycetous fungus were utilized in SCO production of crude glycerol. Overall lipid yield of 0.44 g/g of dry biomass was accumulated, 4 times higher than pure glycerol control, with 38.0% of glycerol conversion throughout the process. A similar trend was also shown by Chatzifragkou et al.
[27], who produced an improvement in lipid yield of 11.6 g/L with 70% of fat in biomass via application of eukaryotic microbes,
Thamnidium elegans, for SCO production. In this study, acetic acid and mannitol were also generated as their side-products.
- 4.
-
Yeast
Succinic, citric, itaconic, and malic acids may be generated from glycerol utilizing fungus of the genera
Rhizopus,
Aspergillus, and
Ustilago [66][67]. However, recently, yeasts also exhibit the promising potential to catabolize crude glycerol via an aerobic route into citric and succinic acid.
Yarrowia lipolytica exhibits the ability in upgrading crude glycerol into citric acid under the aerobic condition with optimal process parameters
[68][69][70]. Li et al.
[71] investigated the effect of co-fermentation of crude glycerol with various agro-residues into succinic acid under aerobic condition using
Y. lipolytica. At the end of the process, 53.6 g/L of succinic acid concentration, process productivity of 1.5 g/L/h, and an overall yield of 0.5 mol/mol were produced.
3.1.2. Bio-Electrochemical Fermentation
Bio-electrochemical fermentation is one of the emerging metabolic pathways which combines both biochemical and electrochemical approaches in upgrading crude glycerol. This technology exploits microbes to catalyze redox reactions in an electrochemical reactor under mild processing conditions
[72]. Microbial catalysts on the electrode have been utilized to enhance the electrochemical reaction as well as increase the rate and yields of glycerol conversion. In this approach, the electric current supplied was utilized to permit the fermentation of crude glycerol as the feedstock. By improving cells’ capacity to regenerate NAD
+ into NADH, the cathodic current will stimulate microbial reduction processes and thus be able to change fermentation profiles. As mentioned in the previous study
[73], a crucial NAD
+/NADH ratio greater than 4 has been linked to high PDO productivity and a high specific growth rate of
K. pneumoniae. However, there are still a very small number of investigations using glycerol bio-electro fermentations within the cathode
[72][73][74][75][76]. Zhou et al.
[72] studied the carbon and electron fluxes during the bio-electro fermentation of crude glycerol using batch biocathodes and showed an increase in 1,3-PDO generation, while in the study by Choi et al.
[77],
C. pasteurianum was used and successfully demonstrated a shift in the microbial metabolism with improved PDO production when an electrical potential is applied. Recently, Xafenias et al.
[74] reported a significant increase in PDO synthesis with aid of
Clostridiaceae. High PDO concentrations of 42 g/L were recorded which shows the potential of this approach to be further studied.
3.2. Thermochemical Approaches
3.2.1. Gasification Pyrolysis
Pyrolysis is a simple method of upgrading glycerol, yet it is just as significant as other processes. This method involves pyrolyzing feedstocks under specific processing conditions—high temperature (>600 °C), high pressure, in the deoxygenated environment inside a continuous or batch reactor
[78][79], resulting from the thermochemical conversion of carbon contained in the feedstock of crude glycerol. The following processes take place to generate gaseous end-products
[80]: (1) pyrolysis and devolatilization of feedstocks at relatively low temperature; (2) further degradation of the primary byproducts by continuous heating; and (3) coking gasification which leads to the production of high value-added syngas including carbon monoxide (CO), ethylene, methane, and hydrogen (H
2).
Dehydration and hydrogenation reactions dominantly happened throughout this approach. In the previous work of Skoulou and Zabaniotou
[81], they used mixed crude glycerol, crude olive oil, and grains in a fixed bed reactor operating at 750–850 °C. Co-gasification attempts were made to increase the amount of hydrogen produced from the gas produced during the gasification process. According to the findings, combining crude glycerol with olive particles at a biomass weight ratio of 49% resulted in gas emissions of between 0.4 and 1.2 Nm
3/kg
[78][81]. In another work, based on
Figure 5, Blass et al.
[82] employed gasification pyrolysis of glycerol with H
2 in a reactor containing dehydration, hydrogenation and upgrading stages in series with help of HZSM-5 and Pd/α-Al
2O
3 catalysts at a temperature of 400 °C to produce a mixture of acetaldehyde, acrolein and hydroxypropanone, propanal, and olefins. Propanal condensed over Brønsted acid sites to produce C
4–5 olefins, which then underwent high conversion to produce C
2–3 olefins, which accounts for this. During this staged process, a C–C bond formed, and negligible carbon was dissipated as CO as a byproduct. Thus, due to environmental concerns of greenhouse gases production, industrial scaleup is limited.
Figure 5. Schematic illustration of three staged reactor used for gasification pyrolysis of crude glycerol feed.
3.2.2. Fast Pyrolysis
Fast pyrolysis is a method that has been adopted to decompose biomass into essential chemical feedstocks and biofuels usually with presence of catalyst. For the manufacture of highly stable liquid fuels, the catalyst-assisted cracking process is executed at temperatures between 400 and 800 °C with a short residence time of between 0.5 and 3 s
[79][81][83]. The yield and characteristics of the end-products of the process are also significantly influenced by different types of feedstocks, environment, and particularly catalyst species
[84][85]. Hence, optimizing the parameters promotes enhanced byproducts yield such hydrocarbon oils with shorter chain (C
1–12), gases, and char
[86][87]. Conventionally, hydrotreating of bio-oils and hydrocarbon wastes, derived from engine oil, transmission oil, and hydraulic oil, which fractured into high value-added products via thermal decomposition of pyrolysis and treated with external hydrogen gas has become a key technology in upgrading bio-oil into transportation fuels
[88]. However, from the researchers' knowledge, there are no works on hydrotreating of crude glycerol which may be due to its high operating costs, and it is not worth it in waste upcycling, in term of its techno economic view.
There are several previous published works on upgrading crude glycerol via fast pyrolysis assisted with catalysts. He et al.
[83] performed catalytic fast pyrolysis of crude glycerol into bio-based BTX in a tandem micro-reactor using ZSM-5/bentonite catalysts which operating at 520–536 °C. The study resulted in only 8.1 wt.% of bio-BTX yield (15% carbon yield) based on crude glycerol feed with fresh catalyst. In their work, the reduced end-product yields are mainly due to coking issues. The majority of the coke (10.5 wt.%) deposit was on the ZSM-5 planes, which not only reduced the number of Lewis and Brønsted acid sites but also clogged the pores, deactivating the catalyst. From previous published works, relatively poor BTX yields (2 wt.%) were also recorded, with different catalysts—Al
2O
3 [89] and Pd-Ru catalysts
[90]. However, in other works
[91][92], zeolites favored the production of acrolein (86%) from catalytic conversion of glycerol with higher glycerol conversion.
3.2.3. Supercritical Fluids
One of the alternative thermochemical conversion techniques for crude glycerol utilizes supercritical fluids, including water, ethanol, methanol, and CO
2, as the processing medium. This method has drawn a lot of interest recently and was demonstrated to be an appropriate reaction medium for biomass reforming. Either with or without the assistance of a catalyst, supercritical fluid reforming of crude glycerol and model compounds was already explored
[9][93][94][95][96][97]. As an additional point, crude glycerol incorporates 6.5% of water content; thus, the prior drying step can be neglected via this hydrothermal approach which reduced its processing cost.
Lighter weight liquid constituents and permanent gases, mixture of H
2, CO, CO
2, methane, and higher hydrocarbons will be produced during this glycerol upgrading process, with or without a catalyst. In addition, the rate of conversion, reaction selectivity, and the nature of the gas obtained are acknowledged to be impacted by the inclusion of a catalyst–homogenous catalysts (metal salt and acid catalysts lead to enhanced glycerol conversion and acrolein production). The utilization of various catalysts within this approach has been discussed in previous literature works by Markocic et al.
[98] and Pavlovic et al.
[99]. With the utilization of catalysts, the energy required for the procedure is reduced and it proceeds at lower operating temperatures and minimizes capital expenditures.
According to previous studies
[94][95][98][100][101], it has been observed that the main solvent for crude glycerol upgrading via supercritical fluid reforming is water, while there are not many works utilizing CO
2 [93], and methanol
[102]. According to Markocic et al.
[98], utilization of water is due to the unique properties of supercritical water itself, when temperature and pressure of the normal water both reached its critical point (T
c = 373 °C, P
c = 22.1 MPa). The solubility of additional molecules of glycerol in water is thus improved by the decreasing dielectric constant, and supercritical water takes on the characteristics of a nonpolar solvent
[103]. Other than that, reduced dynamic viscosity of supercritical water also leads to improved diffusion coefficients of dissolved substances. Therefore, polar, and ionic reactions as well as free radical reactions may occur at this state. In sub- and supercritical water, crude glycerol conversion proceeds quickly, and the high solubility of the intermediates in supercritical water prevents the development of tar and coke, and high product yields are produced at comparatively low temperatures
[100][101].
3.2.4. Steam Reforming
Crude glycerol can be upgraded into H
2 using the steam reforming method. In steam reforming of crude glycerol, there are four main steps—feedstocks refinement, reforming, water-gas-shift (WGS) reaction, and end-product refining
[104]. In the first step of raw material purification, sulfur and chloride impurities are removed. Then, in a fixed-bed reactor, the reforming process is commonly conducted. Thus, the raw material is brought into contact with steam while being catalyzed by a heterogeneous catalyst, resulting in the production of syngas and other gaseous byproducts. The catalytic WGS reactor collects this gas mixture, where the CO combines with the steam to produce more H
2. Finally, high purity H
2 is produced by purifying the resulting gas stream using various techniques, including pressure swing adsorption (PSA) and/or membrane systems. This strategy is suitable to prepare chemicals made of oxygenated hydrocarbons, including waste/bio-glycerol. These processes may be complemented by undesirable ones, which including methanation, dehydration, carbon precursor reactions, and dehydrogenation, depending on the operational circumstances.
Since scaling up the steam reforming strategy to an industrial level would not necessitate substantial changes to the present infrastructures used for natural gas reforming, this method can be regarded as one of the most promising glycerol upgrading technologies
[105]. Industrial steam reforming exploits γ-Al
2O
3 supported Ni catalysts as its catalyst option due to their increased availability and lower cost compared to precious metal-based catalysts
[106][107][108][109][110]. Furthermore, Ni-based catalytic systems are capable of cleaving C–C, O–H, and C–H bonds as well as the WGS reaction, with typical NiO contents of 10 to 25 wt.%
[111]. WGS can eliminate the adsorbed CO from the surface of the catalyst by converting it into CO
2, the first three bonds must be broken in the reforming phase
[106][109]. Due to its improved mechanical and chemical resistance, large surface area (S
BET), and favorable metal dispersion, the γ-Al
2O
3 support is also commonly employed. However, coke deposition and particle sintering cause some deactivation in these systems. The first occurrence is often attributed to the γ-Al
2O
3 acidic characteristic, whereas sintering is attributed, among other aspects, to metal phases and γ-Al
2O
3 hydrothermal disturbances
[106][112]. Within steam reforming operation parameters, these changes are often connected to the transition of γ-Al
2O
3 into other stable phases.
3.2.5. Aqueous Phase Reforming
With a similar objective as steam reforming technology, the catalytic method of aqueous phase reforming was developed to convert oxygenated hydrocarbons generated from biomass into H
2-rich gas. However, when compared to other catalytic thermochemical approaches such as pyrolysis, gasification, or steam reforming, aqueous phase reforming exhibited various advantages
[113][114]. Aqueous phase reforming chemically converts the liquid phase-feedstocks at ambient processing conditions, low temperature, around 150 to 300 °C, due to the partial evaporation of the water. Operating pressure, approximately 1.5–7 MPa, is used during the reformation of crude glycerol, such that ample pressure was exerted to allow the H
2-rich effluent to be in situ filtered via swing adsorption approach or, in some cases, using membrane technology which addresses its storage issue and efficiently isolated CO
2 from the main products. With extremely low CO concentrations (100–1000 ppm), it is possible to generate H
2 gas in a batch reactor assisted with metal catalysts, produced higher amount of H
2 as compared to the existing steam reforming technique. In conclusion, this approach functions at minimal pressure, at a low temperature, and with less costly technology
[104]. Additionally, they are simple to integrate into safe, environmentally friendly energy production systems.
3.2.6. Microwave-Assisted Pyrolysis
The microwave absorbent must be capable of absorbing microwave energy and heat to the requisite temperature in order to pyrolyze waste
[115]. Based on a study by Leong et al.
[116], using a carbonaceous catalyst and temperatures between 300–800 °C, a microwave heating technique utilized to convert crude glycerol collected from biodiesel plants into biofuels. The product yields in each phase of such a process is determined by the processing conditions including residence time, process temperature, and type of catalysts employed during the reaction, all of which affect the reaction system and relative activation energy. From previous studies
[115][116][117][118], it is observed that there was a decreased total mass of gaseous products due to the catalyst’s inclination toward hydrogen gas selectivity. Other than that, temperature reduction and longer residence times increased the overall energy production. The findings demonstrated that crude glycerol has the potential to produce syngas and bio-oil for use in bioenergy
[118].
Figure 6. Microwave-assisted pyrolysis of crude glycerol by magnetron microwave in a quartz reactor with the presence of activated carbon as catalyst.