Antibiotic resistance continues to evolve and spread beyond all boundaries, resulting in an increase in morbidity and mortality for non-curable infectious diseases. Due to the failure of conventional antimicrobial therapy and the lack of introduction of a novel class of antibiotics, novel strategies have emerged to combat these multidrug-resistant infectious microorganisms.
1. Introduction
The rise of antimicrobial resistance urgently requires the development of new strategies for improving antibiotic activity in infected patients, to minimize the risk of the emergence of resistant strains, and to prevent patients’ reinfection and spreading of resistant bacterial strains in hospitals. Regarding the most concerning bacterial pathogens, the ESKAPE group (
Enterococcus faecium,
Staphylococcus aureus,
Klebsiella pneumoniae,
Acinetobacter baumannii,
Pseudomonas aeruginosa, and
Enterobacter species) represent some of the greatest threats to human health due to their ability to evade antibiotic treatments through various mechanisms of resistance
[1]. Thus, it has been recently reported that such infections claimed 5 million lives in the world by 2019, and this trend will only increase in the coming decades with more than 50 million deaths estimated worldwide by 2050 if nothing is done
[2]. Despite the human impact of MDR infections, numerous companies have ceased their antibiotic research programs due to the low level of financial profit generated by these products
[3]. During recent decades, faced with this worrying health concern, an alternate way has appeared, i.e., rejuvenating usual antibiotics to restore their biological efficacy
[4][5][6][7]:
-
By blocking the inactivating enzyme;
-
By tackling the mechanisms that impair the intracellular concentration required for antibacterial activity;
-
By bypassing target mutation.
2. Mechanism of Resistance
2.1. Membrane Impermeability
In Gram-negative bacteria, porins are transmembrane proteins facilitating the transport of hydrophilic molecules across the outer membrane, while hydrophobic molecules can diffuse through the phospholipid bilayer. Consequently, charged antibiotics, such as ß-lactams, will cross the outer membrane by way of the porin in order to reach the periplasmic target
[8]. To limit the antibiotic action, the Gram-negative bacteria have developed mechanisms that decrease their membrane diffusion. Changes in outer membrane permeability impair the influx of hydrophilic antibiotics using porins like β-lactams and fluoroquinolones
[9]. An alteration in the porin expression or in porin integrity leads to a decrease in internal concentration and therefore resistance to these antibiotics. For example,
Klebsiella aerogenes (formerly
Enterobacter) becomes resistant to cephalosporins because of an amino acid mutation located inside the eyelet of porin that strongly reduces the pore diameter. This structural mutation generates a restricted diffusion of antibiotics through porins, conferring this peculiar resistance
[10].
2.1.1. Antibiotic Target Modification
The antibiotic affinity on a target is a particularly important event during the fight against a bacterial infection. A target modification caused by spontaneous mutation at the gene level (substitutions of amino acids on binding sites located on the target surface), alters the affinity of the antibiotic, favoring the emergence of a resistance level
[11]. The involvement of mutations in the QRDR region in a quinolone target, gyrase and topoisomerase enzymes, is extensively documented in Gram-negative pathogens
[12].
2.1.2. Antibiotic Inactivation
Numerous enzymes produced by bacteria can inactivate the antibiotic either by modifying or hydrolyzing it. Thus, these enzymes can act against β-lactams, aminoglycosides, chloramphenicol, or antibiotics belonging to the macrolide-lincosamide-streptogramin (MLS) family
[13]. Inactivation of antibiotics occurs either by hydrolysis or by association with modifying metabolism or elimination (phosphorylation, acetylation, etc.). The β-lactam antibiotics exhibit a β-lactam ring which is the effective part of the molecules, as it inhibits the transpeptidase involved in the synthesis of the Gram-negative bacterial wall; the hydrolysis of this part is one of the degradation mechanisms involved in antibiotic resistance. Thus, the bacterium is able to produce enzymes, termed β-lactamases, able to hydrolyze the β-lactam ring
1 [14][15].
2.1.3. Efflux Pumps
Efflux pumps are transmembrane systems by which the cells expel toxic compounds outside using active transport (energy-dependent), contributing to the bacterial resistance mechanism. A decrease in internal antimicrobial amount under the biological required concentration impairs and limits the antibiotic action
[16]. The efflux pumps are classified into six families: the ABC (Adenosine triphosphate-Binding Cassette) family, the MFS (Major Facilitator Superfamily) family, the MATE (Multidrug And Toxic Compound Extrusion) family, the SMR (Small Multidrug Resistance) family, the RND family (Resistance Nodulation cell Division), and finally the PACE family (Proteobacterial Antimicrobial Compounds Efflux)
[17].
2.2. Strategies to Circumvent Gram-Negative Bacterial Resistance
One of the most promising approaches to circumvent Gram-negative bacterial resistance requires the combination of several types of molecules (e.g., enzyme inhibitor + antibiotic or membrane permeabilizer + antibiotic), for which the biological action can be either additive or synergistic. The additive effect occurs when the observed effect is the sum of the activity of the two partners, while the synergy corresponds to a final action greater than the sum of the activity of the two individual partners
[18]. The use of this combination can also minimize the risk of the emergence/spreading of bacterial resistance for the treatment of recurrent infections
[19].
2.2.1. Drug Combination
To restore the activity of an antibiotic impaired by a resistance mechanism, an effective strategy consists in associating two antibiotics during patient treatment. The development of the hybrid antibiotic approach needs a chemical covalent connection between two molecules; generating a single hybrid drug that targets bacterial cells can mitigate bacterial resistance mechanisms. In this context, a combination of two antibiotics, such as a fluoroquinolone (ciprofloxacin) and an aminoglycoside (neomycin B), demonstrated a significant delay in resistance emergence compared to each component taken alone, and this is thanks to the multiple antibiotic resistance operon “Mar” involved in the regulation of efflux pump expression and OM permeability [20].
In the multiresistant (MDR) bacterium Pseudomonas aeruginosa, it has been shown that the hybrid tobramycin-moxifloxacin 3 led to better intracellular concentration of antibacterial molecule by (i) increasing the outer membrane permeability and (ii) altering the PMF (proton motive force) which is the energy-driven force of efflux pumps involved in the expulsion of toxic compounds (metabolites, drugs, antibiotics, etc.). Therefore, the combination of moxifloxacin 1 with tobramycin 2 allowed a weaker development of resistance compared to each antibiotic alone (Figure 1) [21].
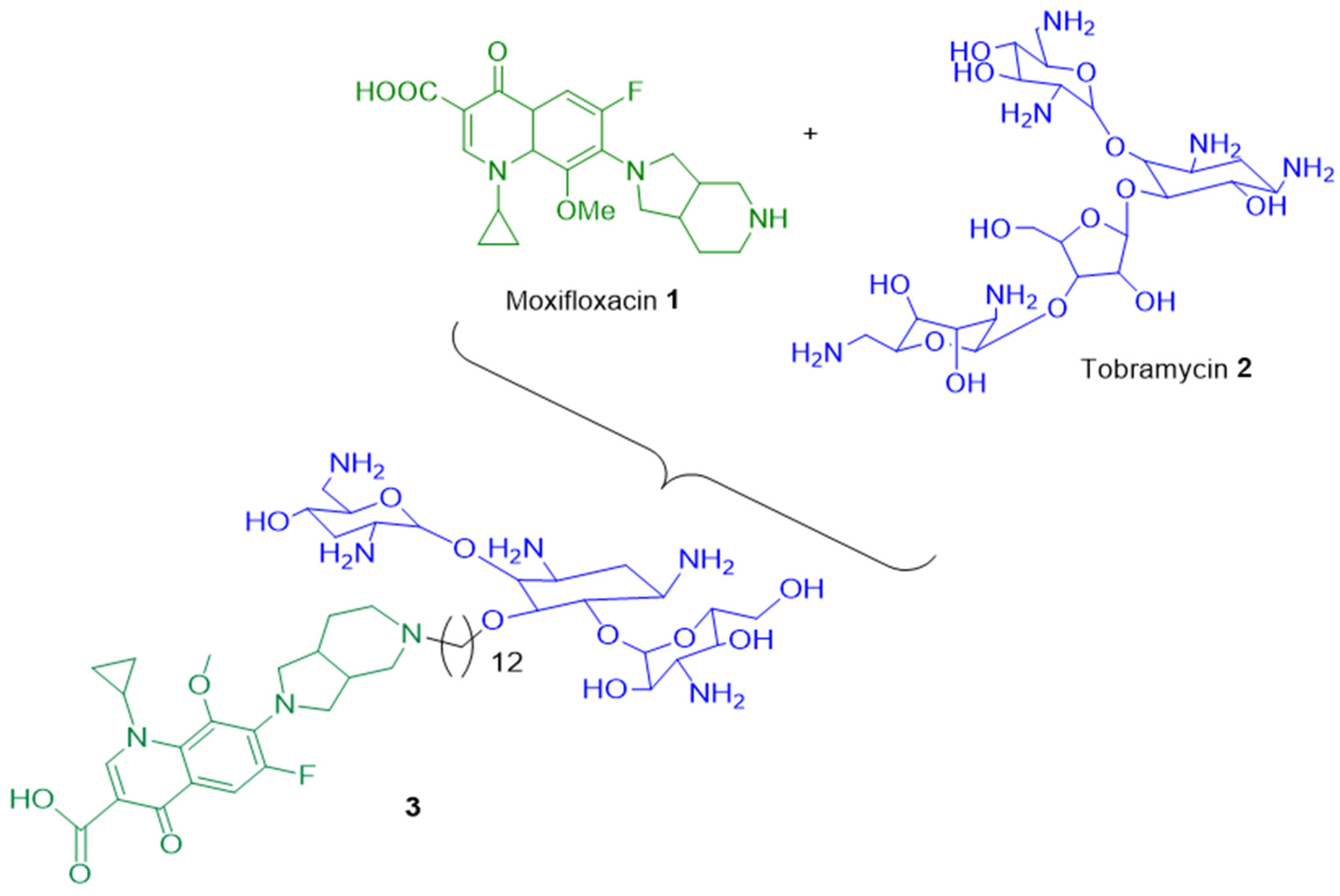
Figure 1. Drug combination 3 of moxifloxacin with tobramycin.
2.2.2. Antibiotic and β-Lactamase Inhibitor Combination
β-lactam antibiotics used in combination with a β-lactamase inhibitor (
Figure 2) constitute a pioneering and efficient strategy to combat β-lactam resistance. Thus, the amoxicillin/clavulanic acid combination, also known as co-amoxiclav or amox-clav and sold under the brand name Augmentin
®, is a medication used for the treatment of several bacterial infections. Amoxicillin
4 is a semi-synthetic penicillin, and clavulanic acid
7 is a β-lactamase inhibitor, both possessing a similar half-life
[22]. They are mainly used for the treatment of acute otitis media
[23], lower respiratory tract infections, urinary tract infections, and other infections due to bacteria resistant to amoxicillin alone
[24]. The effect of this combination limits the maximum daily dose of amoxicillin administered orally
[22], but on the other hand, clavulanic acid has a limited effect on antimicrobial resistance, expands the spectrum of amoxicillin, and therefore results in problems in gut health by increasing side effects
[25]. In addition, the sulbactam
8–ampicillin
5 (or cefoperazone) combination restores the activity of this antibiotic through the β-lactamases’ inhibition and also presents an inherent activity against certain
A. baumannii strains, which is the advantage for such a combination. However, this combination did not show strong selective pressures for enterobacteria that produce β-lactamases and enterococci that are resistant to vancomycin. It is mainly used for the treatment of urinary, nosocomial, gynecological, and intra-abdominal infections and cellulitis
[26].
Figure 2. Structure of β-lactam antibiotics 4–6 and β-lactamase inhibitors 7–10.
Numerous Gram-positive and negative bacteria are resistant to β-lactams due to three potent factors: (1) alteration of the outer membrane in
P. aeruginosa and
Enterobacteriaceae species, (2) low affinity of PBPs (penicillin-binding proteins) in
N. gonorrhoeae and
P. aeruginosa, (3) destruction of the β-lactam ring by periplasmic β-lactamases in aerobic Gram-negative bacteria, such as
E. coli and
Klebsiella species
[27]. Combination of this class of antibiotics with β-lactamase inhibitors renders these bacteria more susceptible. Thus, the β-lactamase enzyme is inhibited by β-lactamase inhibitors which bind irreversibly to the enzyme, preventing its action on the β-lactam ring and restoring its antimicrobial activity
[28].
2.2.3. Efflux Pumps Inhibitors
Efflux pumps expel the antibiotics towards the extracellular environment and contribute to bacterial resistance. Some are selective and only expel specific substrates, while others are non-selective and expel various classes of antibiotics and non-specific substrates (detergents, organic solvents, dyes, etc.)
[29]. In this case, a possible strategy consists of blocking efflux systems by so-called efflux pump inhibitors (EPIs) and therefore restoring the susceptibility of resistant strains. Several methods are used to target efflux pumps: (1) inhibit the expression of genes encoding these pumps, (2) deplete the pumps of the energy necessary for their functioning, (3) compete in the inner membrane transporter, (4) block the outlet channel in the outer membrane, (5) prevent the assembly of pump components at the membrane level
[30]. For example, the use of a natural steroidal alkaloid extracted from the antidysentery plant
Holarrhena, conessine
11, demonstrated a reduction in the minimum inhibitory concentrations of various antibiotics including tetracycline, cefotaxime, levofloxacin, novobiocin, rifampicin, and erythromycin and an inhibitory activity of MexAB-OprM efflux pumps (
Figure 3)
[31]. Epigallocatechin-3-gallate (EGCG
12) extracted from tea is another compound responsible for inhibiting this type of efflux pump in
P. aeruginosa [32].
Figure 3. Structure of efflux pumps inhibitors 11–16.
Reserpine
13 is an antipsychotic drug extracted from the alkaloid plants
Rauwolfia serpentina targeting the efflux pumps of the superfamilies MFS and RND by directly interacting with amino acid residues in the efflux transport protein of tetracycline antibiotic. This plays another role against
S. aureus by increasing 4-fold the activity of norfloxacin released by the NorA pump. Piperine
14, which is another alkaloid, is able to inhibit this NorA efflux pump and then enhance ciprofloxacin accumulation in
S. aureus [33].
Quinoline derivatives such as pyridoquinolones
15 act as competitive inhibitors of the AcrAB-TolC efflux pump and restore norfloxacin activity against
P. aeruginosa. Additionally, the amide dipeptide compound PaβN
16 is one of the first EPIs discovered through a chemical approach. It inhibits the efflux pumps of the RND family and increases the activity of many antibiotics such as fluoroquinolones, macrolides, and phenicols, but on the other hand, it presents a toxicity towards mammalian cells, suggesting a limited therapeutic use
[34][35].
2.2.4. Liposomes Addressing Resistance
The liposomes adhere to the bacterial membrane (outer membrane) and contribute to the release of the antibiotic into the periplasmic space (
Figure 4)
[19]. The comparison between the Minimum Inhibitory Concentration (MIC) values of the antibiotic (tetracycline) present in a solution and in a liposome shows an important decrease (MIC in the solution is equal to 0.063 and 0.125 μg/mL for
S. aureus and
S. epidermidis, respectively, whereas there is a 3.9-fold decrease for
S. aureus and 12.8-fold for
S. epidermidis when the antibiotic is present in the liposome). This reduction indicates that the liposomes decrease the antibiotic doses required due to the fusion of the liposome structures with the bacterial membranes according to the mimetic characteristics
[16][17].
Figure 4. Fusion of liposomes to microbial cell membranes and release of antibiotic.
2.2.5. Potentiation of an Antibiotic Activity in the Presence of Natural Organic Compounds
Natural organic compounds, such as flavonoid polyphenol curcumin
17, can also be used to potentialize antibiotic antibacterial activity (
Figure 5). Thus, this led to the restoration of the bactericidal activity of oxacillin and norfloxacin against resistant
S. aureus (MRSA) and linezolid activity against
Mycobacterium abscessus by permeabilizing the membrane, altering cell viability and thus facilitating the penetration of the antibiotic. It is used for the treatment of tory bowel syndrome, diabetes, and asthma in Asian medicine
[36].
Figure 5. Structure of curcumin
17 and carvacrol
18.
2.2.6. Potentiation of an Antibiotic Activity in the Presence of Inorganic Compounds
Inorganic compounds including metals, ceramics, glass ceramics, and polymers can act as antimicrobial agents through several mechanisms: alteration of membrane function, production of reactive oxygen species (ROS), release of toxic ions, and loss of oxygen enzyme activity. Their size, shape and concentration can have a great impact on their antimicrobial activity
[19].
These size- and structure-dependent properties rely on the ability to synthesize and modify materials below 100 nm, ranging up to 200 nm for use in medicine and pharmaceutical products, such as metal nanoparticles (NPs) and metal oxide nanoparticles; NPs are useful due to their chemical and mechanical stability, control of morphology, and particle size
[37].
AgNPs are involved in three mechanisms to inhibit bacterial physiology, as illustrated in Figure 6.
Figure 6. Mechanisms to alter bacterial physiology by using AgNPs. (1) Membrane permeabilization, (2) Interaction with DNA, (3) Liberation of ROS, (4) Interaction with cellular components.
The first acts on membrane permeabilization: AgNPs penetrate the periplasmic space, and its adhesion to the internal membrane induces the destabilization of the bacterial cell and leakage of the cell content, leading to the death of the bacteria.
The second mechanism deals with the affinity between AgNPs and sulfur or phosphorus present in the intracellular medium at the level of DNA and proteins; an alteration of the respiratory chain present in the inner membrane could occur due to the affinity of AgNPs towards thiol groups of enzymes, inducing the release of reactive oxygen species (ROS), resulting in damage of the cellular machinery and activation of the apoptotic pathway.
The third mechanism consists in the interaction of the silver released by the nanoparticles with cellular components, which can lead to the modification of the metabolic pathways and alteration of the membrane and of the genetic material
[38].
In another field, dressing is used to heal severe wounds, but by contamination, it impedes healing due to the competition between cells and bacteria on the wound surfaces. For a wound dressing to be ideal, it must preserve cell attachment to regenerate tissue and have an antibacterial effect. The use of methylcellulose (MC) foams alone against
E. coli and
S. aureus has no effect on their growth; however, its association with Manuka honey (MH) MC-MH produced by freeze-drying shows a clear reduction in growth. Meanwhile their association with B3 (dense borate ion), based on bioactive glass (BG) doped with copper (MC-MH-B3-BG-Cu), shows a much more effective antimicrobial effect compared to MC-MH, the bacterial viability of
E. coli decreases from 60% to 0%. Copper is an antibacterial ion that penetrates the bacterium and leads to the degradation of its DNA. Therefore, MC-MH-B3-BG-Cu foams can be used as a wound dressing for the treatment of infected wounds
[39].
3. Outer Membrane Permeabilization
The outer membrane (OM) of Gram-negative bacteria constitutes an additional permeability barrier which protects the cell from many more antibacterial agents compared to Gram-positive bacteria. The negatively charged components of the OM interact with permeabilizers, promoting the entry of impermeable molecules. Permeabilizers are considered as potential antibiotic adjuvants to fight against bacterial resistance [29][40]. Pentamidine, an anti-protozoan drug, has shown effective activity against a wide range of Gram-negative bacteria by interacting with the lipopolysaccharide, and as a result, disrupting the outer membrane. In addition, the cationic peptide and its derivatives destroy the outer membrane by electrostatic interaction.
Menadione, a soluble synthetic vitamin converted into vitamin K2 at the intestinal level, was used to see its effect on membrane permeability against multiresistant bacteria such as S. aureus, P. aeruginosa, and E. coli. It has been demonstrated to have an antibacterial activity only against the P. aeruginosa bacterium, but when combined with antibiotics of the aminoglycoside family, it produces a synergistic effect and decreases the MICs for these antibiotics [41].
Polyamines (
Figure 7) also have a role in the fight against bacterial resistance. The two quinoline polyamine derivatives
19 and
20 are used as an adjuvant for doxycycline by improving its activity against
P. aeruginosa. A disruption of integrity and depolarization of bacterial membranes have been observed with derivative
20, while the mechanism of action of derivative
19 is not yet known
[42]. The polyamine farnesyl
21 to
24 derivatives make the bacterium
P. aeruginosa sensitive to the antibiotic tetracycline, and this is linked to the hydrophobicity of the antibiotic and to the alteration of the integrity of the bacterial outer membrane
[43][44]. In addition, the polyamine derivatives motuporamine
25 and
26, extracted from the marine sponge
Xestospongia exigua, are used as adjuvants for doxycycline; they have an antibacterial effect by modifying the transmembrane electrical potential, which results in an alteration of proton hemostasis
[45].
Figure 7. Polyamine derivatives 19–26.
4. Cargo Delivery: Conjugation of Antibiotics with Siderophores or Sugars (The “Trojan Horse” Strategy)
4.1. Siderophores
Siderophores are low-molecular-weight metabolites produced by microorganisms and plants involved in iron capture. They have a strong affinity for iron, compete with iron transporters in the host body, and belong to pathogen virulence factors
[31][46]. When the availability of iron is limited in the environment, bacteria synthesize and secrete siderophores to scavenge iron and transport it inside the bacterial cell. Two types of siderophores exist: endogenous siderophores synthesized by the bacterium itself and exogenous siderophores synthesized by other microorganisms “cross-feeding”
[47][48][49].
The use of iron transporters (siderophores) could be an interesting alternative to circumvent the obstacle presented by the Gram-negative bacteria envelope during the entry of many antibiotics. Thus, the construction of antibiotics linked to siderophores can facilitate the penetration of the antibiotic in the cytoplasm. Gram-negative bacteria import Fe3+ in the form of ferric siderophore complexes across the outer membrane by TonB-dependent transporters (TBDTs). This translocation depends on the complex composed of the ExB/ExbD/TonB proteins present in the bacterial membrane
[50].
Siderophores can be used as vectors, allowing the vectorized antibiotic to easily pass through the bacterial membrane, reach the target, and therefore reduce the phenomenon of resistance. This strategy consists of connecting a linker between the siderophore and the antibiotic (
Figure 8). This linker must be variable in length and chemical properties depending on the target, stable in the extracellular environment, and hydrolysable in the bacterial cell
[51].
Figure 8. Antibiotics conjugated with siderophores.
Albomycins are sideromycins secreted by the bacterium
Streptomyces; their siderophore part is composed of ferrichrome, the serine is the linker, and the antibiotic is composed of a thioribosyl-pyrimidine which inhibits the enzyme seryl t-RNA synthetase and therefore the protein synthesis
[52]. In
E. coli, peptidase N cleaves the linker between the siderophore and the antibiotic. If the pepN gene coding for this peptidase is mutated, the albomycin loses it biological activity and transports iron into the bacterial cell. It is used in the treatment of various bacterial infections, so this strategy will help the use of albomycins as new antimicrobial agents, even if it is more effective against Gram-negative bacteria than Gram-positive bacteria
[53].
Interestingly, pyoverdine has an amine group in its structure, which allows it to bind to the antibiotic via a linker; the antibiotic is ampicillin, which is a β-lactam. Conjugates 27 and 28 (Figure 9) tested against ampicillin-resistant P. aeruginosa ATCC27853 and ATCC13692 have reported high antibacterial activities, with MICs ranging from 0.01 pg/mL to 0.67 pg/mL, respectively.
Figure 8. Pyoverdins conjugates 27–28.
Pyochelin is also used in the Trojan horse strategy as a cargo approach (
Figure 10). C5 of pyochelin is modified, and then two analogs are synthesized,
29 having a triple bond and
30 possessing a single bond (softer aliphatic chain)
[54]. The conjugation of the terminal amine group of pyochelin with the antibiotic norfloxacin against
P. aeruginosa ATCC1592 cultured in succinate medium (starved in iron level) shows that the conjugates
31 and
32 bearing a non-hydrolysable succinic linker do not present any antimicrobial activity at a concentration of 10 μM. However, this conjugation, made this time by a hydrolysable linker
33 and
34 at the same concentration, shows a high antimicrobial activity and a high inhibition of bacterial growth.
Figure 10. Pyochelin conjugates 31–34.
Nowadays, a highly targeted Trojan horse strategy has emerged with cefiderocol
35 (
Figure 12). Its structure contains a catechol-type siderophore fused to antibiotic “cephalosporin”, this hybrid being active against Gram-negative resistant bacteria like
P. aeruginosa,
A. baumannii Enterobacteriaceae, and
S. maltophilia [55]. It has received approval for use from the United States Food and Drug Administration (FDA).
Figure 11. Structure of cefiderocol 35.
Once cefiderocol is in the periplasm, iron is released, and the antibiotic binds to penicillin-binding proteins (PBPs) and then inhibits peptidoglycan synthesis, resulting in cell death (
Figure 12). It is stable against ESBL β-lactamases (extended-spectrum β-lactam), AmpC, and carbapenemases and active against class A, B, C, and D β-lactamases. Its activity against
A. baumannii is more potent than ceftazidime–avibactam and meropenem against enterobacteria-producing carbapenemases (KPC) resistant to meropenem. This activity is the same or slightly higher than ceftazidime–avibactam against
K. pneumoniae [56].
Figure 12. Mechanism of action of cefiderocol 35.