Boronic ester, similar to the imine or acylhydrazone bond, has been widely applied to construct COFs. The boronic esters serving as the COFs’ linkers are formed through the condensation of boronic acids and catechol derivatives. Introducing fluorescent units modified with such boronic acids and catechol derivatives, the luminescent COFs can be constructed. In 2008, Wan et al. reported the first example of a belt shaped luminescent COF, consisting of a typical fluorophore pyrene and triphenylene functionalities
[36]. Utilizing the solvothermal method, the COF was synthesized through a condensation reaction of 2,3,6,7,10,11-hexahydroxytriphenylene and pyrene-2,7-diboronic acid. The COF could harvest a wide range of photons, due to the intramolecular singlet energy transferred from the triphenylene to the pyrene units, and showed bright luminescence. Since then, a series of luminescent COFs linked by boronic ester bonds have been reported. For example, the McGrier group constructed three luminescent COFs containing a homogeneous and heterogeneous distribution of dehydrobenzoannulene (DBA) vertex units
[37]. These COFs were synthesized by condensation reaction of the pyrene-2,7-diboronic acid and different ratio of catechol modified DBA. The three COFs were named after Py-DBA-COF 1, Py-DBA-COF 2, and Py-MV-DBA-COF, respectively. Py-DBA-COF 1 and Py-DBA-COF 2 were formed via linking PDBA with DBA
[12] and DBA
[38], respectively, while Py-MV-DBA-COF was obtained by condensing PDBA with DBA
[12] and DBA
[38], in which the molar ratios of PDBA, DBA
[12], and DBA
[38] were 2:1:1. The Py-DBA-COF 2 showed a blue-greenish luminescent color and Py-DBA-COF 1 and Py-MV-DBA-COF were yellow emissive. Compared to the DBA
[38]-based COFs, the lowest-energy transitions of the DBA
[12]-based COFs were symmetry-forbidden in the ground state, which could be the reason why the emissive colors of these COFs were different. Besides polycyclic aromatic hydrocarbons like pyrene, AIEgens are also charming fluorophores with which to construct boronic-ester-bond-linked luminescent COFs. The Jiang group reported the first AIE-based COF which was formed by condensation of TPE-cored boronic acid and 1,2,4,5-tetrahydroxybenzene
[39]. Incorporating TPE units into such COFs which possessed rigid environment could reduce the excitation-energy dissipation of the TPE units, resulting in high luminescence of the COF with the absolute fluorescence quantum yield up to 32%. In this regard, boronic ester bond can be considered a feasible linkage to binding more emissive elements for fabricating a series of luminescent COFs.
5. Polymeric Emissive Materials Based on Transesterification
Transesterification is a typical reversible reaction where an ester can transform into another through an associative and dissociative process of the alkoxy moiety and it has been widely used in laboratories and industries
[40] (
Scheme 4). In order to facilitate the transesterification reaction, elevated temperature as well as a catalyst are both necessary factors. In 2011, Leibler and coworkers developed a new kind of polymeric material which was later named vitrimer
[41]. The vitrimer, composed of epoxy networks, could rearrange its topology and show viscosity variation through transesterification mechanism. After that, increasing attention has been attached to transesterification for exploiting more polymeric materials with ideal characters
[42]. Nevertheless, emissive dynamic polymers based on transesterification reactions have rarely been reported. Recently, a blue emissive self-healing polymer composed of polyglycerol and itaconic anhydride was synthesized by Shan et al. and the fluorescence intensity of corresponding polymers could be selectively quenched by Fe
3+ [43]. However, in this system there are no regular relationships between the transesterification and the fluorescence behavior of the polymer. As mentioned above, the transesterification reaction could significantly affect the mechanical performance of the polymers. Therefore, it is meaningful to realize the visualization of the transesterification in the polymer system. The Cui group reported a dynamic polymer based on transesterification which showed fluorescent color exchange as the transesterification reaction took place
[44].
Scheme 4. The representative reaction formula of transesterification.
6. Polymeric Emissive Materials Based on Diels–Alder Reaction
The Diels–Alder (DA) reaction, named after Diels and Alder, was first discovered in 1928
[45]. It refers to a [4+2] cycloaddition reaction where an electron rich diene and an electron deficient dieniphile as the reactants form a cyclohexene product (
Scheme 5a). The DA reactions are generally reversible in nature and the retro DA reactions usually require elevated temperature to take place. The cycloaddition between funans and maleimides (
Scheme 5b) is one of the most representative DA reactions, in which the equilibrium moves forward at room temperature but backward at about 110 °C
[46]. Besides funans and maleimides, the DA reaction can also take place between π-extended anthracene and maleimide derivatives to form five-membered ring products which are more stable under elevated temperature compared to the cycloaddition products of funans–maleimides
[47]. To cleave the DA bonds of anthracene–maleimide derivatives, or in other words, to trigger the retro DA reaction in anthracene–maleimide derivatives, the temperature usually needs to be heated to 200 °C. External force, similar to elevated temperature, can also trigger the retro DA reaction of anthracene–maleimide derivatives in a polymer system (
Scheme 5c). The DA bond, as a fundamental linkage, was used to construct polymer networks dating back to 1979
[48]. Since then, numerous polymers based on DA bonds have been reported. The reversibility of the DA reaction has made it the earliest chemical method to fabricate dynamic covalent polymers with a self-healing property
[49]. By embedding fluorescence moiety into the DA-based polymer, stimuli-responsive emissive materials can be developed.
Scheme 5. (a) The general formula of reversible Diels–Alder reaction. (b) Elevated temperature-induced reversible DA reaction between furan and maleimide derivatives. (c) Force-induced retro DA reaction between π-extended anthracene and maleimide derivatives.
Due to the easy formation under mild conditions and the reversible property at elevated temperature, DA bonds have been used to develop various kinds of thermal-stimuli-responsive luminescent polymers. In 2017, Mutlu et al. reported a thermally driven self-reporting polymer released system
[50]. In this system, pyrene units were modified to parent polymers through DA reactions. There were some nitroxide radicals which could quench the luminescence of the pyrene units linked to the polymer chains. Thus, the emission of the polymer was silent under ambient condition. When treated with elevated temperature, the pyrene units were released from the polymer, resulting in the fluorescence enhancement of this system and the fluorescence intensity maxima was located at 397 nm. Additionally, the Martin group prepared several DA based π-conjugated polymers and studied their thermal-triggered optical properties in detail. First, they grafted oligo-(phenylene ethynylene)s (OPEs)-based π-conjugated donor and acceptor to two polymer chains via DA reaction, respectively
[51]. Mixing these two polymers and heating up to 67 °C for several days, the mixed donor–acceptor grafting polymer was obtained. Owing to the fluorescence resonance energy transfer from the donor to the acceptor, the side chain grafting polymer showed efficient emission of the acceptor even if excited by absorption maximum of the donor. They further introduced the π-conjugated donor and acceptor into the polymer main chain
[52].
Anthracene can be seen a diene to react with suitable dienophiles (maleimide derivatives), forming [4+2] DA cycloadducts
[53]. During the formation process of the cycloadducts, the conformation of the anthracene transforms from the planar into twist state. When external force is applied to the adducts, the retro DA reaction will occur and facilitate the anthracene to return to the planar π-extended state which is emissive nature. Due to the mechanoluminescence, anthracene–maleimide, acting as the polymer linker sensitive to mechanical force, has been gradually studied
[54][55][56]. In 2016, linear poly(methyl acrylate) (PMA) and crosslinked poly(hexyl methacrylate) containing anthracene–maleimide were developed by the Sijbesma group
[57]. In the work, the π-extended anthracenes could be released from the anthracene–maleimide adducts via applying mechanical force to the polymer, both in the solution and solid state, and further showed blue emissive feature. Hence, the fluorescent π-extended anthracene releasing system could be used as a sensitive probe for mechanical force. They further introduced triplet–triplet annihilation photon upconversion (TTA-UC) into the mechanical sensing system
[58]. 9-phenylethynylanthracene, a known emitter in TTA-UC systems, was modified to PMA and then linked to a maleimide-group-terminated PMA through DA reaction. Adding the triplet sensitizer octaethylporphyrin to the as prepared poly(hexyl methacrylate), a mechanical force induced TTA-UC emission of the 9-phenylethynylanthracene could be detected and the upconversion quantum yield was 0.0823%. Very recently, the Li group adopted the same strategy to prepare a mechanical sensing polymer showing two distinct emissions
[59]. These two emissions derived from the anthracene and amino-substituted maleimide, respectively. The maleimide fluorophores exhibited fluorescence quenching in protic or polar solvent, enabling dual emission behavior from this single polymer system in different solvents. All these DA-bond-linked polymers demonstrated above showed mechanical trigging emission behavior which might be applied to detect the damage of functional polymers. It is interesting that the dynamic break and reform of the DA bond can adjust the conjugation, even the electron distribution of the polymeric material, which is superior to other dynamic bonds, such as imine and boronic ester bonds, for regulating the optical properties of polymers.
7. Polymeric Emissive Materials Based on Dynamic C-C Bond
C-C bonds are generally regarded as irreversible and stable. However, the triphenylmethyl dimer itself was found that could undergo break and reform reaction via reversible recombination and homolytic cleavage of a C-C bond to form triphenylmethyl radicals
[60], which, facilitating the dynamic C-C bond. Actually, the dynamic C-C bonds refer to reversible δ
(C-C) bonds (
Scheme 6a) which can undergo association and dissociation reaction under mild condition without catalysts. For achieving the dynamic C-C bond, the stabilization of the radical state through promoting spin-delocalization is significant. Introducing bulky substituents to protect the spin center and extending the π-systems to participate in the spin delocalization have proved to be effective methods
[61][62]. As most DCBs, dynamic C-C bonds have been used as the reversible linkers of the polymers to build versatile dynamic covalent polymers. Diarylbibenzofuranone (DABBF), a typical molecular containing central dynamic C-C bond (
Scheme 6b), has been widely incorporated into the polymer structures
[63]. When the C-C bonds homogeneously cleave under stress, the blue radical derived from diarylbibenzofuranone can be obtained. There are some similar molecules that can also show color change behavior as their dynamic C-C bonds are cut off, such as tetraarylsuccinonitrile (TASN) (
Scheme 6c), diarylbibenzothiophenonyl, difluorenylsuccinonitrile and diarylbiindolinone, etc.
[64][65][66][67]. TASN, the unique one among these mechanochromic molecules, could present a luminescent behavior when transformed into corresponding radicals, which was first reported by the Otsuka group.
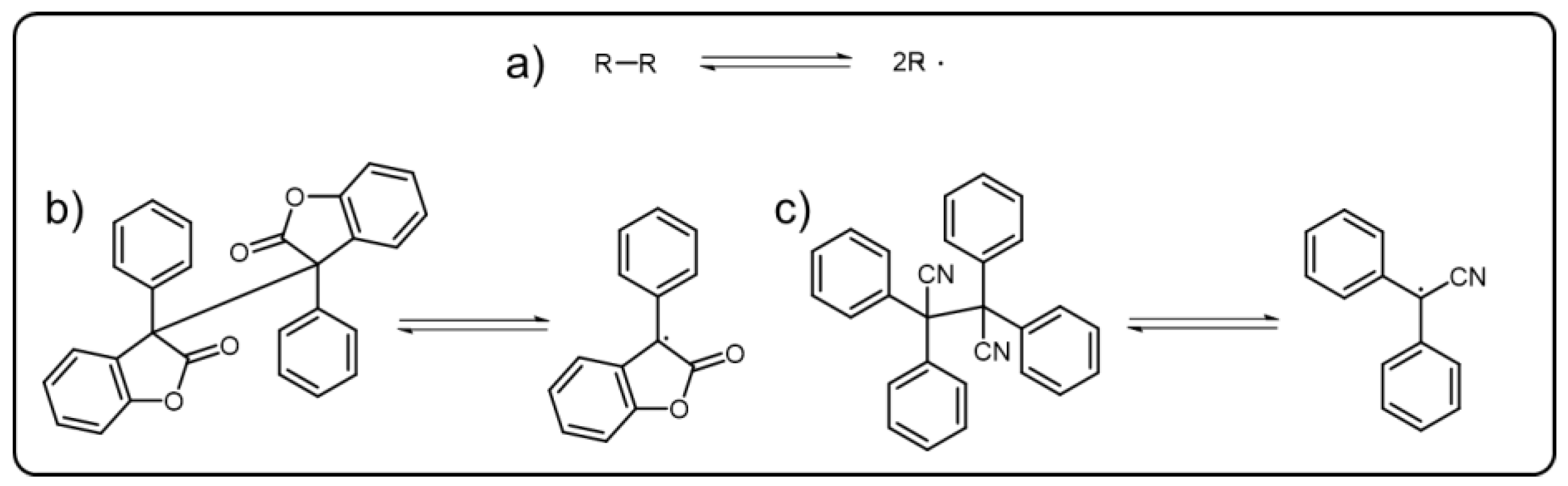
Scheme 6. (a) Schematic representation of reversible dynamic C-C bond. The typical central radicals derived from (b) DABBF and (c) TASN.