2. Tumour Immunity Cycle
Our immune system is a complex system that operates through two main modalities of response: innate immunity (a non-specific and quick response) and adaptive immunity (subsequently activated antigen-specific response that mediates immune memory)
[11].
Although a number of cellular components are involved in this complex system, antitumour immune surveillance is based on T lymphocytes, as demonstrated by several mice models, in which the loss of T-cell activity was associated with uncontrolled tumour growth. T lymphocytes recognise and identify some molecules released from cancer cells as foreign (or “antigens”). These tumour-associated molecules are mutated proteins, showing alterations caused by the high genetic instability of tumours. Indeed, the high degree of genetic diversity in cancer cells accelerates their evolutionary fitness but also increases the divergence from a normal cell, facilitating recognition by the immune system. The frequency of non-synonymous somatic mutations per DNA megabase in the coding genome of a tumour is called “tumour mutational burden” (TMB)
[12][13].
Overall, activating antitumour immunity is a multistep process involving several lymphocyte populations. The uncontrolled growth and the consequent high TMB of cancer cells firstly result in the activation of innate immunity, including natural killer (NK) cells, which target cancer cells and favour their apoptosis leading to the release of tumour-associated antigens. These molecules are released into the tumour microenvironment (TME) and are subsequently recognised by antigen-presenting cells (APCs), which capture these neo-antigens and present them to the corresponding T-cell receptor (TCR) of naïve T lymphocytes via the major histocompatibility complex (MHC).
The final step of these immune events is the release of co-stimulatory cytokines by CD4+ T cells and the activation of antigen-specific CD8+ T cells leading to the lymphocyte-mediated destruction of tumour cells (
Figure 1)
[14][15].
Figure 1. Exemplification of tumour immunity cycle. Natural killer and other cells of the immune system provoke lysis of tumour cells with liberation of tumour peptides. These peptides are exposed on surface molecules on dendritic and other antigen-presenting cells and are recognised by CD4 T lymphocytes (priming phase). These lymphocytes, therefore, acquire the ability to identify tumour cells and to promote a cytotoxic response via CD8 lymphocytes (effector phase). MHC: major histocompatibility complex; TCR: T-cell receptor; CD: cluster differentiation; CTLA-4: cytotoxic T lymphocyte-associated protein 4; PD-1: programmed death-1; PD-L1: programmed death 1-ligand.
3. Tumour Immune Escape
In acute infections, T cells can eliminate the pathogen. Instead, in the case of advanced tumours, the neo-antigen is rarely eliminated and, consequently, a chronic inflammatory stimulation is established with the possibility of losing effectiveness. In fact, chronic immune stimulation activates negative feedback mechanisms (in particular, the activation of immunosuppressive cells that silence the immune response) and promotes a progressive loss of the cytotoxic capacities of T cells.
On the other hand, cancer cells can develop a series of resistance mechanisms aimed at evading the host’s immune control. Namely, tumour cells can acquire the ability to express molecules that inhibit the immune response or reduce the expression of the MHC complex in APCs
[16][17]. The most known immune checkpoint molecules are CTLA4, PD-1 and its ligand PD-L1, and lymphocyte-activation gene 3 (LAG-3). All of them are receptors expressed on the membrane of T cells in different phases. They counterbalance lymphocyte activation in physiological conditions to avoid unnecessary tissue damage, chronic inflammation, and uncontrolled lymphocytic proliferation.
In detail, CTLA-4 is expressed by T cells in the priming phase, exerting inhibitory actions following interaction with its ligands, CD80 (B7-1) and CD86 (B7-2), which are expressed on the surface of the APCs. PD-1, instead, is expressed in the effector phase, especially under conditions of chronic exposure to the antigen, and exerts an inhibitory action on the lymphocytes via interaction with its ligands, PD-L1 and PD-L2, found in the TME. Similarly, LAG3 is expressed in lymphocytes during the effector phase
[18]. LAG3’s most important ligand is represented by the MHC class II molecules expressed on the surface of APCs, with an interaction ultimately leading to T-cell exhaustion
[19].
Tumours can exploit these mechanisms to avoid immune-mediated destruction not only by expressing ligands activating the immune checkpoints themselves but also favouring the development of a tolerant TME through the recruitment of non-neoplastic cells expressing these ligands.
Immune checkpoint inhibitors (ICI) are monoclonal antibodies specifically designed to disrupt these ligand/receptor interactions, removing the inhibition of T cells and promoting their antitumoral cytotoxic activity (
Table 1)
[20][21][22].
Table 1. Immune checkpoint inhibitors classified according to their main targets.
CTLA-4: cytotoxic T lymphocyte-associated protein 4; PD-1: programmed death-1; PD-L1: programmed death 1-ligand; LAG3: lymphocyte activation gene-3.
4. Mechanisms of Primary ICI Resistance
4.1. Tumour-Intrinsic Mechanisms
Tumour intrinsic mechanisms include a lack of tumour immunogenicity (low TMB, heterogeneous antigens, mutation of critical genes involved in immune regulation), defective antigen presentation, and aberrations in several signalling pathways (Figure 2).
Figure 2. Overview of tumour-intrinsic mechanisms of resistance to immune checkpoint inhibitors. MHC: major histocompatibility complex; TCR: T-cell receptor; CD: cluster differentiation; PD-1: programmed death-1; PD-L1: programmed death 1-ligand.
Low TMB (currently defined as <10 muts/Mb, using a pan-cancer threshold)
[12][13] is one of the principal mechanisms of primary resistance to immune therapies. Tumours with the highest number of somatic mutations, being enriched in neo-antigens, are therefore potentially more immunogenic. Accordingly, the best response rate to ICIs can be observed in tumours with high TMB, such as melanoma and non-small cell lung cancer (NSCLC). In patients bearing these cancers with high TMB, ICI treatment was associated with an improved overall survival (OS), while patients harbouring neoplasms with low TMB showed a lower objective response rate to ICIs and a poorer OS
[23][24][25]. However, most patients did not respond to immunotherapy regardless of their TMB status, suggesting that the presence of high tumour somatic mutations is only one factor contributing to the immune response.
Another mechanism of ICI resistance is the dysfunction of neo-antigen presentation. As previously stated, tumour cells produce neo-antigens that can be recognised by the MHC system. To avoid identification by T cells, tumour cells can decrease the expression of neo-antigens, such as by the hypermethylation of genes expressing these antigens
[13]. Moreover, the activation of anti-cancer-specific CD8+ T cells requires an efficient presentation of tumour antigens. Cancer cells can develop mutations in genes associated with MHC presentation, such as beta-2 microglobulin, leading to the severe dysfunction of the antigen-presenting mechanism and resistance to ICIs
[26][27][28].
Loss-of-function mutations in the Janus tyrosine kinases 1 and 2 (JAK-1 and JAK-2) is another cause of intrinsic resistance to ICIs. The integrity of these kinases is essential for interferon-alfa (IFN-α) intracellular signalling. Since the INF-α pathway plays a vital role in the priming of T cells by APCs, a defective JAK1/2 pathway efficiently inhibits the activation of effector T cells
[8][10].
Additionally, the WNT/β-catenin pathway promotes the resistance to ICIs by the transcriptional repression of key chemokine genes, leading first to a depletion of basic leucine zipper ATF-like transcription factor 3 (Batf3)-lineage DC recruitment, and then to a consequent failure to prime and recruit CD8+ T cells
[29].
The WNT/β-catenin pathway is of particular interest in the case of HCC, as approximately 40% of these tumours show the constitutive activation of WNT/β-catenin signalling induced by relevant gene mutations
[30][31]. Most interestingly, constitutive WNT/β-catenin activation had negative effects both on the disease control rate and progression-free survival (PFS) of HCC patients who received anti-PD1 therapies
[32][33].
The phosphatase and tensin homologue-signal transducer and activator of transcription 3 (PTEN-STAT3) pathway is another crucial pathway in immune regulation. PTEN knock-down decreases the ability of T cells to kill tumour cells through the indirect activation of STAT3, a transcription factor that is a key signalling node and a regulator of the critical hallmarks of cancer, tumour angiogenesis, resistance to apoptosis, metastasis, and immune evasion
[34]. In particular, STAT3 activation has been linked to inhibitory effects on immune response, including the down-regulation of triggering receptor expressed on myeloid cells-1 (TREM-1) and the reduced expression of Toll-like receptors and interferon-inducible genes
[35]. Nearly 60% of human HCCs exhibit activated nuclear STAT3, and STAT3 activation is associated with a poor prognosis
[35].
4.2. Tumour-Extrinsic Mechanisms
Tumour-extrinsic mechanisms are mainly due to the immune-suppressive effect of TME. They include primary T-cell-related factors (activation of alternative immune checkpoints, T-cell exhaustion and phenotype alteration), immunosuppressive cells, cytokines and metabolites released into the TME (
Figure 3). As the TME includes immune cells, blood vessels, fibroblasts, signalling molecules, and the extracellular matrix surrounding tumour cells, many factors can contribute to the development of an immune-suppressive TME
[36].
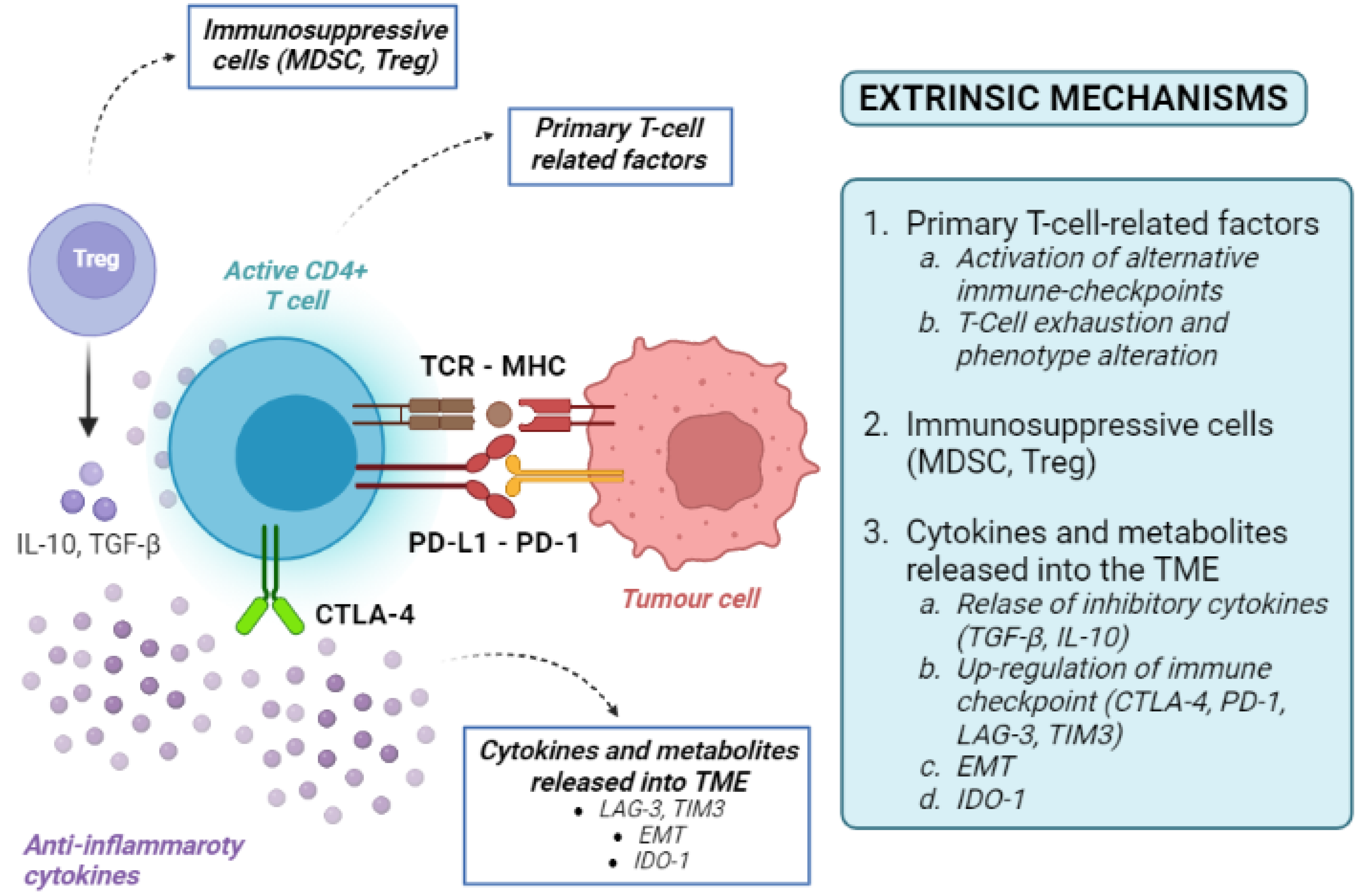
Figure 3. Overview of tumour-extrinsic mechanisms of resistance to immune checkpoint inhibitors. MHC: major histocompatibility complex; T-reg: regulatory T cells; TCR: T-cell receptor; CD: cluster differentiation; PD-1: programmed death-1; PD-L1: programmed death 1-ligand; MDSC myeloid-derived suppressor cells; IL-10: interleukin-10; CTLA-4: cytotoxic T lymphocyte-associated protein 4; TME: tumour microenvironment; EMT: epithelial-to-mesenchymal transition; TGF-β: transforming growth factor-beta; IDO-1: indoleamine-pyrrole 2,3-dioxygenase 1.
Infiltration of the TME by unique types of immune cells, including myeloid-derived suppressor cells (MDSCs) and regulatory T cells (Tregs), can suppress T-cell recruitment and responses. MDSCs and Tregs are both immune cells, but derive from different lineages (myeloid and lymphoid, respectively). Under physiological conditions, both populations are involved in the modulation of immune response, thereby maintaining homeostasis and self-tolerance
[36][37]. This aim is achieved through the release of inhibitory cytokines (i.e., transforming growth factor beta—TGF-β—or IL-10) or the up-regulation of immune checkpoints (such as CTLA4 and PD-1)
[36][37]. Alternative co-inhibitory immune checkpoints on T cells, including T-cell immunoglobulin, can also be up-regulated, inducing T-cell exhaustion by activating the apoptosis pathways
[9]. High tumour infiltration by MDSCs has been correlated with poor prognosis and resistance to ICI therapy, as they can release metabolic enzymes inhibiting T-cell expansion and promoting the conversion of naïve T cells into Tregs
[36].
LAG-3 is an example of the activation of immune checkpoints outside of the classical PD-1/PD-L1 axis. Its expression has been associated with a poor prognosis in patients with HCC
[38]. Furthermore, T-cell immunoglobulin and mucin-containing protein-3 (TIM-3) and its ligand galectin-9 can activate a complex cascade, ultimately leading to T-cell exhaustion
[38]. LAG-3, TIM-3 and PD-1 can act synergistically, facilitating HCC immune evasion and mediating the resistance to classical PD-1/PD-L1 blockades
[39][40].
Under different circumstances, TME-mediated resistance to ICIs might not depend on immune-derived cells primarily. Epithelial-to-mesenchymal transition (EMT) is a cellular process enabling epithelial cells to gain mesenchymal features leading to an aggressive and motile phenotype
[41]. EMT can promote an immunosuppressive TME via the recruitment of APCs, inducing tolerance, up-regulation of the immune checkpoint, and resistance to NK cell-mediated lysis
[42][43]. The association between EMT, immunosuppression, tumour progression and metastasis has been reported in different cancer types, including HCC
[44].
On most occasions, a very complex interplay between immune and non-immune cell resistance is the cause of resistance. The VEGF and TGF-β pathways are master regulators of these processes. The VEGF signalling pathway is a well-known regulator of neo-angiogenesis production by HCCs. It can also produce immune resistance via the induction of the Fas ligand, resulting in the death of tumour-infiltrating CD8+ T cells
[45]. TGF-β is a multifunctional cytokine with a multitude of effects on immune and stem cell differentiation and regulation
[46][47]. In many cancer cells, the disruption of TGF-β signalling causes the simultaneous proliferation of both cancer cells and the surrounding stromal cells in an immunosuppressive and pro-angiogenic microenvironment
[48]. Additionally, TGF-β can convert effector T cells into regulatory T cells
[49] and exert inhibitory effects on B cells
[50], turning off the inflammatory reaction and favouring tumour immune escape.
Last but not least, even the composition of extracellular matrix molecules can influence the recruitment of immune-suppressor cells or exclude T-cell infiltration. In breast cancer studies, T cells easily migrated in a loose collagen matrix, while a dense collagen matrix hampered T-cell migration
[51][52]. Indoleamine-pyrrole 2,3-dioxygenase 1 (IDO-1) is a heme-containing enzyme in the extracellular matrix that can interfere with immune clearance. IDO-1 is physiologically expressed in many tissues and cells, but its activation can also help malignant cells escape immune clearance
[36]. Tumours with high IDO1 can deplete the essential amino acid tryptophan from the TME, resulting in T-cell anergy and immune suppression
[53].
5. Mechanisms of Acquired ICI Resistance
Although the specific mechanisms of acquired (secondary) resistance are poorly understood, some of these are overlapped in primary and acquired ICI resistance. In the specific case of HCC, these mechanisms are still elusive.
Tumour cells can lose some encoding sequences for tumour neo-antigens through the elimination of specific sub-clones or the deletion of chromosomal regions, resulting in the selection of less immunogenic tumour cell clones spared from T-cell killing
[54][55]. For example, an acquired homozygous loss of beta-2 microglobulin, decreasing the expression of HLA and disrupting the antigen presentation, was identified in lung cancer. Defective HLA class I antigen processing through deleterious mutations in beta-2 microglobulin has been shown in melanoma
[56][57]. Regarding melanoma, acquired resistance to PD-1 inhibitors can also be mediated by JAK1/2-inactivating mutations.
The up-regulation of both immune checkpoints and immune-suppressive cytokines may be other causes of acquired resistance. For instance, lung cancer patients with acquired resistance against anti-PD1 therapy show an up-regulation of PD-1
[9]. Although it is still unclear whether PD-L1 expression causes an increased or decreased ICI response, it could result in the suppressed antitumour effect of T cells
[58].
6. Current Strategies in Immunotherapy for HCC
The initial studies of immunotherapy in HCC patients included ICI monotherapies
[59]. Despite promising results of Phase 2 trials, two different Phase 3 randomised clinical trials failed to show the superiority of nivolumab vs. sorafenib in the frontline setting, and pembrolizumab vs. placebo in the second line setting, respectively
[60][61]. Nevertheless, trials of ICI monotherapies provided three pieces of important information: First, these regimens were safe and well-tolerated, even in patients with liver cirrhosis and chronic viral infections. Second, ICIs obtained an objective response rate in 15–18% of cases
[60][61], which is considerably higher that of TKI. Third, patients with an objective response had very long survival times
[62].
Therefore, associating ICIs with agents able to overcome resistance to immunotherapy has become the most common strategy to improve the response rate and survival of HCC patients (Figure 4).
Figure 4. Overview of current therapeutic strategies to overcome resistance to immunotherapy. MHC: major histocompatibility complex; TCR: T-cell receptor; CD: cluster differentiation; PD-1: programmed death-1; PD-L1: programmed death 1-ligand. CTLA-4: cytotoxic T lymphocyte-associated protein 4; TME: tumour microenvironment; LAG3: lymphocyte-activation gene 3; TIM3: T-cell immunoglobulin and mucin domain 3; VEGF: vascular endothelial growth factor; TLR9: Toll-like receptor-9; mTKIs: multitarget tyrosin kinase inhibitors; TGFb: transforming growth factor-beta.