Plant performance can be improved under adverse environmental conditions by integrated soil–water–plant solutions or integrated soil fertility and plant nutrient management. Microorganisms living in the soil, the rhizobiome, are distinguished from endophytes living inside plants.
1. Plant Performance in the Field
Plants are sessile organisms. In the field, they have to cope with some fluctuating environmental factors. Therefore, plants have a certain window of resistance that allows for instant adaptation to the respective spectrum of environmental factors
[1], with different resistances among plant species. In several experiments, it was observed that a narrow tolerance window correlates with a high growth rate, while broadening the window of resistance will result in reduced growth rates
[2][3][4]. Therefore, it may be expected that at locations with only little fluctuation in environmental factors, and rare incidents of extreme weather conditions, the most abundant plant species may be characterized with a narrow window of resistance
[5]. Several of our crops are characterized with a narrow window of resistance as compared with their wild ancestors, since breeding programs were aimed at high-yielding crops. In contrast to earlier breeding concepts, more recent projects are aimed at breeding for crop accessions that will produce a reliable yield, even under slightly suboptimal growth conditions
[6][7][8][9][10].
Fluctuating illumination on a cloudy day, day–night changes, etc. are environmental changes that each plant has to tolerate. Plants will preferentially adapt by the regulation of enzyme activities rather than the production of new enzymes and modification of enzyme abundance. Therefore, these responses can occur almost instantly and easily escape detection in the field. Accordingly, most of the experiments that analyze these immediate responses were carried out in laboratories
[11]. In general, these short-term events will not cause immediate damage, but may affect the resource use efficiency. On the other hand, long lasting periods of drought, heat, lack of nutrients, soil salinization, etc., will adversely affect plant performance at a significant degree. These conditions have been termed persistent stress. In accordance with Larcher
[12], researchers have to distinguish between (i) mild stress, which allows stressed plants to thrive and subsequently re-adjust to a new physiological equilibrium, and (ii) severe, lethal stress, which is beyond the resistance level of the respective plant species (see
Table 1).
In the literature, environmental stress is often referred to as abiotic stress to differentiate it from biotic stress, which is caused by pathogens and herbivores. With respect to its global economic importance, abiotic stress is estimated to cause 50 to 80% of yield losses in crop production
[13][14]. The calculation is based on a comparison between the current annual crop yield and theoretical yield. This can be performed by scientists under completely controlled conditions in a greenhouse
[15]. Therefore, the precise extent of yield reduction can be questioned. Nevertheless, the enormous economic impact of abiotic stress is beyond any discussion.
Plant response to abiotic stress is described as a multifactorial trait
[16]. Moreover, plant species differ to a large degree in the preferential use of response patterns. This applies to any type of abiotic stress. Drought and salt stress are of primary importance for agriculture in arid areas. However, these two stresses can be found in other climatic zones, as well. Accordingly, many scientists have focused on analyzing the effects induced by the application of these two types of stress. The common trait between both stresses is that plant roots can sense low levels of available water. Therefore, some of the plant responses to these two types of abiotic stress are quite similar, such as osmolyte production (sugars, amino acids, and other organic molecules), in which the osmotic potential in plant roots adjusts to facilitate water uptake from the soil. In the case of soil salinity, some plants use an import of inorganic ions rather than osmolyte synthesis (on the expense of assimilate consumption) to control cellular osmotic potential
[17][18][19].
Table 1. Plant response to environmental stress.
All types of stress will affect the physiology of a plant as a whole. This indicates that stress perception is followed by signaling events (
Figure 1). In the case of water shortage and salinity, ABA is one of the messengers. However, reactions other than closure of stomata and abscission of leaves can take place as a response to abiotic stress, as well. Among the stress responses, major physiological processes not under ABA control, such as nitrogen fixation, respiration, photosynthesis, and carbohydrate metabolism are included
[25].
Figure 1. Environmental stress and observed concepts of stress adaptation.
From the description of ecosystems, it was evident that plant species differ to a large degree with respect to environmental preferences. In addition, this applies to the stress resistance level of plants. For instance, a puzzling observation was that subsequent to salinization a new plant population with a different frequency of species occupied abandoned areas. These highly salt-resistant species are referred to as halophytes
[26][27][28]. Respective plant species turned into experimental plants in many research teams. The idea was to compare sensitive and resistant plant species to better understand concepts of stress perception, signaling, and responding. This approach requires the standardization of methods to allow for a comparison of the results. This need was addressed in a handbook that provides standardized protocols for the measurement of plant features, which reflect the ecological strategies of species
[29]. After 10 years, the handbook has been updated to provide protocols on traits and methods that had proven to be useful in laboratories as well as in field studies. Furthermore, the new handbook contains additional protocols for functional traits of organs apart from leaves
[30].
The traits described in the two handbooks were selected to predict long lasting effects at the ecosystem level. In addition, they might be used for prediction of annual crop yield, although remote sensing methods are preferentially used for these aspects. Moreover, the described standardized methods can be used to monitor the success of stress resistance breeding. However, they do not provide direct information on potential breeding targets at the metabolic and molecular level. Therefore, an impressive number of publications on genome, proteome, and metabolome analyses of plant species and accessions that differ in their stress resistance are available in the current literature
[31][32][33]. Regulation of gene expression during the phase of stress adaptation has been analyzed, and the importance of individual genes for expression of a certain resistance feature has been analyzed by targeted mutation of experimental plants
[34][35][36].
This was very helpful in the research of stress response, in which significant differences could be detected in accessions of the same plant species when harvested from areas with different local climates, soil conditions or the availability of water
[37]. However, even when using plants as different as Arabidopsis and maize, differences in chromatin modification have been found
[38][39].
Notably, the term stress does not exclude positive implications. Moderate stimuli have a positive impact (eustress), whereas excessive stimuli have a negative impact (distress) on plant response. Moreover, the positive effect of extra compounds as different as sugars, amines, elevated CO
2 concentration, hormones, H
2O
2, UV radiation or nanomaterial has been tested in a concentration-dependent manner
[40][41][42][43][44][45]. Several researchers reported that a repetitive application of moderate stress (with or without the addition of compounds) can modify metabolism, fluidity of biomembranes, the content of compatible solutes and ROS scavenging antioxidants, and enzymes
[46]. Furthermore, the parameter value of the stress resistance window of treated plants correlated with the content of these beneficial compounds. Based on these observations, it is a matter of ongoing discussion, whether improved plant performance under stress in the presence of symbionts relies on similar regulatory mechanisms. In the meantime, economic interest has dramatically increased by the fact that (i) metabolites of pharmaceutical interest were among the compounds overproduced under eustress, and (ii) green algae and higher plants responded similarly to the applied eustress
[47].
2. Interaction of Plants and Microorganisms
2.1. General Observations
In the field, plants, fungi, and bacteria form a well-structured community of organisms
[48][49][50][51]. The microbial community of plants is called phyto-microbiome
[52]. Microorganisms living in the soil, the rhizobiome, are distinguished from endophytes living inside plants. As growth conditions vary to a large degree between individual plant organs, specific populations of endophytic microorganisms can be found in each one
[53]. Moreover, with respect to future agricultural application, beneficial microorganisms and pathogens have to be considered separately
[54].
Research was hampered by the fact that most microorganisms of the phyto-microbiome cannot be cultured in vitro
[55][56]. Therefore, information on species numbers and controlling effects, which are exerted on the microbial community by an individual plant genotype as well as the respective developmental stage of plants, was gained only when metagenomic methods became available
[57][58][59]. Beneficial effects exerted on plant performance by rhizobia and mycorrhiza were observed early, and turned into a target of investigations
[60][61] (
Figure 2a). While it was possible to observe rhizobia-induced nodulation and the anatomy of mycorrhiza in the microscope, information on the interaction between plants and their microbial partners were mainly of indirect type. However, it was found that inoculation of low-fertility soil with plant growth promoting bacteria (PGPB) results in an increased production of biomass, an improved stress resistance, and particularly a reduced sensitivity to incidents of drought stress
[45][62][63].
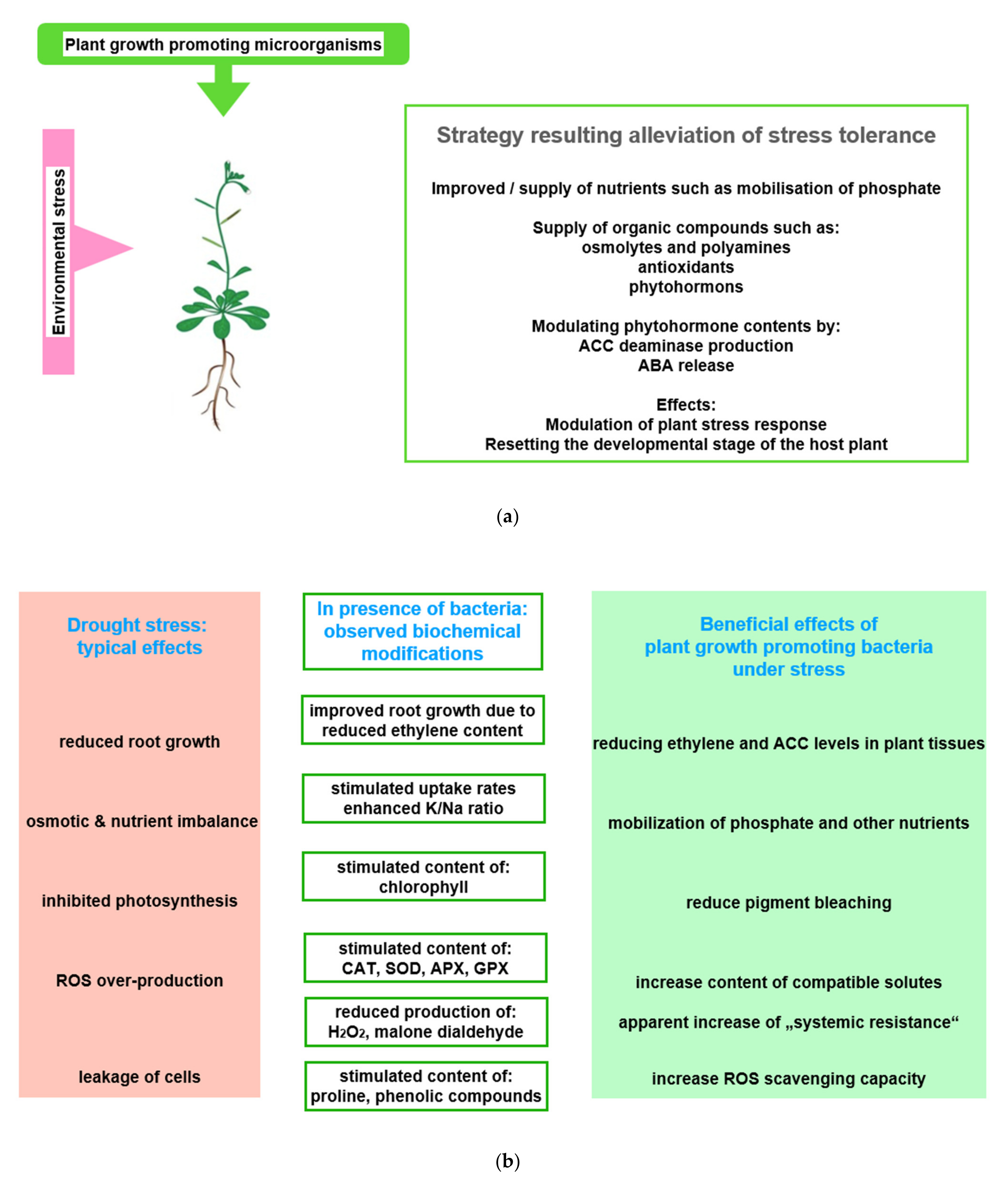
Figure 2. Beneficial effects of plant growth promoting microorganisms (PGPMs). (
a) Observed stress ameliorating effects of PGPMs: The figure symbolizes the service offered by PGPMs, which helps in hosting plants to improve stress tolerance; (
b) physiological basis of observed effects: Using drought stress as an example, typical stress effects are listed in the first column. Column two shows how PGPMs modify the biochemical stress response at biochemical level. In column three, the stress responses ameliorated by PGPMs are listed; (
c) how PGPM activities interfere with the development of plant stress response: The boxes symbolize how PGPM activity may integrate into the reaction sequence, leading to plant stress tolerance. Abbreviations: APX: Ascorbate peroxidase; CAT: Catalase; GPX: Glutathione peroxidase; SOD: Superoxide dismutase.
In this context, for instance, the degree of beneficial effects was observed to be correlated with the improvement of nitrogen use
[64][65]. Recently, in projects that rank the success of inoculation with PGPB, the concentration of intermediates of the TCA cycle, the Calvin–Benson cycle, and photorespiration, which easily could be measured in a laboratory, proved to be useful parameters [
145]. The concentration of these compounds and antioxidants significantly increased in response to osmotic stress
[66]. The modulation of these metabolite concentrations, among others, and the alteration of beneficial enzyme activities were interpreted as possible reasons for improved plant growth (
Figure 2b). Among the more visible symptoms are enhanced seed germination, an expanded and elongated root system, and an increased chlorophyll content. All of the listed parameters are indicators of plant species-specific mechanism, which relieve the adverse effects of abiotic stress
[67][68].
However, data concerning the TCA cycle can sometimes be misinterpreted and misleading. For instance, antioxidants will be degraded under prolonged and intensive stress. However, when monitored over time, the concentration will show a maximum and this maximum will be shifted in the presence of PGPB
[69]. While overproduction of these compounds is preferentially achieved by stimulation of plant metabolism, it is assumed that increased concentrations of metabolites of the indol pathway are based on the import of precursors delivered by PGPB. This applies for shikimic, quinic, and salicylic acids
[70]. The latter compound functions as a messenger in the hosting plant.
2.2. Acquisition of Symbionts
When a plant faces unfavorable conditions, it re-shapes physiological and biochemical parameters, allowing for a modified plant–microbe and microbe–microbe interactions
[71][72]. Plants adapt to the patterns and abundance of suitable species of the rhizo-microbiome by the release of various metabolites, such as signaling molecules as well as organic C and N sources for microorganisms to feed on
[50][52][71][73][74][75][76][77]. The composition of compounds released by the plants can vary with both, the growth conditions and the developmental stage of an individual plant species
[78]. The amount of released carbohydrates may resemble 10% of the assimilate production
[79]. This explains why the plant rhizosphere contains a significantly higher number of microorganisms as compared with the same soil type in the absence of any plants
[80]. The spectrum of microbial species attracted to the plant roots will vary accordingly
[81][82][83]. Moreover, it has been observed that the service of the attracted rhizo-microbiome is fine-tuned to the needs of the hosting plant
[84][85][86][87][88]. Correspondingly, a modified abundance of microbial species occurs in the root-free soil areas and in samples collected from the root surface, respectively.
In response to compounds released by the plant, microorganisms release signaling compounds and metabolites that are beneficial to plants
[78]. This signaling interplay is coordinated between both partners during adaptation to changing environmental conditions
[89][90] (
Figure 2c). This symbiosis can lead to the segregation of other organisms. Some invasive plant species are capable of modifying the abundance of beneficial microorganisms in the soil in favor of organisms that preferentially support the invading species
[88].
2.3. Two Examples of Improved Nutrient Supply Provided by Plant Growth Promoting Microorganisms
As previously described, triose phosphates are exported from the chloroplast exclusively in exchange for phosphate. Therefore, a lack of phosphate inhibits the supply of the plant with assimilates, and indirectly prevents the use of absorbed light energy in photosynthesis. Nitrate reduction is performed using reducing equivalents and ATP. The primary product of nitrate assimilation is glutamine. Lack of water and salinity inhibit growth and the need for assimilates. An excess of energy arises, manifested as an over-reduction of the electron transport chain. As a result, a good nitrate supply can relieve the system by discharging ATP and reducing equivalents for nitrate reduction. In fact, it has been found that PGPMs can improve the plant supply of phosphate and nitrate.
2.3.1. Mobilization of Plant-Inaccessible Phosphate
Nutrient supply and use efficiency of crops have received increased attention due to the disappearance of plant available mineral resources and raw material stocks. Therefore, inoculation experiments using plant growth promoting bacteria in combination with conditions for an efficient nutrient use and minimal nutrient losses, were a promising approach to ensure a sustainable nutrient supply. The expectation was an improved nutrient uptake of the plants and growth promoting effects
[91][92][93]. The PGPM inoculation leads to changes in the root architecture. This effect was explained by the release of phytohormones, such as IAA to the bacteria hosting plants
[94][95][96].
Fertilization with the macronutrient phosphorus is one of the most important strategies to improve crop yield. However, only monobasic (H
2PO
4−) and dibasic (HPO
42−) phosphates are resorbed by plant roots (Perez-Montano et al., 2014 [xx]) and a significant portion of soil phosphorus is unavailable for plants
[97][98]. Phosphate solubilization capability of soil microorganisms is an important feature for plant growth enhancement under moderate fertility conditions
[97][99][100][101]. Based on their ability to secrete organic acids, acid phosphatase, and alkaline phosphatase, phosphate-solubilizing bacteria are able to lower the pH in the rhizosphere, and in this way, increase the soluble and plant available phosphate fraction
[102][103][104]. Notably, as abovementioned, these phosphate-releasing bacteria promote plant growth by offering further compounds in addition to mobile phosphates.
2.3.2. Support of Nitrogen Uptake and Fixation
Few plant growth promoting bacteria species are able to improve nitrogen use efficiency. They are not only involved in N cycling in the rhizosphere, but can also enter plant tissues and modify plant anatomy
[105]. Transfer to plant tissues includes incorporation into seeds, thus promoting the development of next generation seedlings
[88]. As these bacteria lack the nifH gene, enhanced nitrogen assimilation will occur through mechanisms other than nitrogen fixation
[106]. Their bacterial activity correlates with plant species-specific polyamine production
[107]. Interestingly, it was observed that the patterns of accumulated polyamines, amino acids, and urea in an experimental plant was modified by the application of stresses, independent of the growth promoting bacteria species present in the test
[108][109].