Melanoma is the most aggressive and deadly skin cancer. Its incidence has increased steadily in the last decades, especially in the Caucasian population, posing a heightened challenge to the global healthcare system
[1][2]. Relevant geographical variations exist, depending on the clinical phenotype, the genetic background of individuals, and the extent of ultraviolet (UV) radiation exposure
[3]. Currently, it is one of the most frequent cancers in fair-skinned people, especially those with blond or red hair, who have light-colored eyes. Unlike other solid tumors, melanoma mainly affects young and middle-aged people
[4]. Melanoma-related mortality has increased in parallel with the increase in the incidence rate over the years, reaching a mortality rate of one in four deaths
[5]. Nevertheless, the therapeutic landscape of unresectable stage III and IV melanoma has been revolutionized by immunotherapies and targeted therapies. Both strategies have shown markedly improved survival compared with the use of chemotherapy (ChT) regimens
[6]. Melanoma mortality has decreased significantly since the US Food and Drug Administration (FDA) approved ipilimumab in 2011, the first immune checkpoint inhibitor (ICI) to improve survival in the advanced setting
[7][8], and vemurafenib, a v-raf murine sarcoma viral oncogene homolog B1 (
BRAF) tyrosine kinase inhibitor, first in class
[9][10].
2. Molecular Pathways of Melanoma Development
Cancer results from uncontrolled cellular growth of malignant tumor cells caused by a combination of genetic alterations that lead to neoplastic transformation and escape from the inhibitory signals. Several steps in this process are known as the hallmark of cancers
[17].
Several key molecular pathways have been discovered to be involved in the onset, proliferation, survival, progression, and invasion. In this section, researchers summarize the major signaling pathways that are currently known to be dysregulated and involved in melanoma disease.
2.1. MAPK Pathway
Melanomagenesis occurs after mutational events that produce signaling pathways critical for cell survival. Mitogen-activated protein kinase (
MAPK) is a signal transduction pathway, involved in a variety of physiological programs, such as cell proliferation, differentiation, development, migration, apoptosis, and transformation, and is the most relevant in the development of melanoma (
Figure 1)
[18]. The
MAPK pathway is activated by the binding of a growth factor to a receptor tyrosine kinase (RTK) on the cell surface and stimulates the guanosine triphosphatases (GTPase) activity of
RAS. The signal propagates through the RAF, mitogen-activated protein kinase kinase 1 (
MAP2K1), and extracellular signal-related kinase (
ERK) cascade, which enters the nucleus to activate transcription factors and promote the cell cycle (
Figure 1)
[18].
Figure 1. Melanoma key signaling pathways.
The MAPK, PI3K, and NFκB pathways intersect significantly in melanoma pathogenesis. Briefly, in the MAPK-ERK pathway, stimulation of GPCR results in activation of PLC. This promotes DAG and then activates PKC, which stimulates the MAPK pathway. Receptor tyrosin kinases (RTKs) are activated by binding of extracellular growth factor ligands and activate the tyrosine kinase activity of the cytoplasmic domain receptor, starting the cascade of signals. Activated RAS activates the protein kinase activity of RAF isoforms (RAF1, BRAF, ARAF). Each RAF isoform possesses a distinct capacity to activate MEK, with BRAF being the strongest activator. MEK phosphorylates and activates downstream proteins, such as ERK1 and ERK2. ERK can translocate to the nucleus and phosphorylate different transcription factors, which leads to the control of cell cycle progression. MITF is a target of ERK and controls the production of the pigment melanin, cell cycling, and survival. The binding of the ligand to KIT (SCF) results in activation of the MAPK and PI3K pathways. In the PI3K-AKT pathway, ligand binding to the RTK leads to dimerization and autophosphorylation of the receptor and activation. Activated RTK recruits PI3K to the plasma membrane. PI3K activates AKT, whereas PTEN antagonizes this process. PI3K may also be activated by GPCR, IGF-1R, and RAS. Both ERK and AKT activate the mTOR-signaling pathway, which mediates cell survival and proliferation. In the TNFR pathway (canonical NF-κB pathway), binding of the TNF-alpha cytokine to its receptor TNFR1 results in TAK1 activation. TAK1 leads to the aggregation of a downstream kinase complex, the IKK complex. Phosphorylation of IκB by the IKK complex results in the release of NFκB. NFκB translocates to the nucleus and activates genes involved in cell survival and anti-apoptosis.
Fourteen
MAPKs have been identified in mammals, and these kinases are typically divided into three main subfamilies:
ERKs, c-Jun N-terminal kinases (
JNKs), and
P38 kinases. Each of these
MAPKs is activated through phosphorylation by an
MAPK kinase (
MAP2K), which in turn is activated by an
MAPKK kinase (
MAP3K)
[18]. The
ERK pathway is the best-characterized
MAPK pathway, which has a relevant role in the development and progression of melanoma. On this
MAPK axis, the role of
MAP3K is played by the
RAF family of serine/threonine kinases, which is characterized by an RAS/GTP-binding domain. RAS proteins vHa-ras Harvey rat sarcoma viral oncogene homolog (
HRAS),
NRAS, and v-Ki-ras2 Kirsten rat sarcoma viral oncogene homolog (
KRAS) are small GTPases located in the plasma membrane that act as activators in several pathways, apart from
MAPK. Additionally, activation signals via
RAS on the inner surface of the cell membrane increase
ERK activity. Consequently, there is an increase in cellular proliferation, greater cell survival, and resistance to apoptosis. Activated
ERK can also induce the metastatic potential of melanoma through the expression of integrins that promote tumor invasion
[19].
In melanoma, dysregulated
MAPK signaling and sustained
ERK activation can eventually lead to cascade hyperactivity and subsequent cell proliferation, survival, invasion, metastasis, and angiogenesis. The
BRAF gene is frequently mutated in several cancers, and
BRAFV600 is the most common mutation of the skin. Mutated
BRAFV600 leads to elevated
BRAF kinase activity and sustained activation of downstream targets, in addition to unresponsive negative feedback mechanisms
[20]. The mutant
KRASQ61, the most frequent mutation of
KRAS in melanoma, leads to an important decrease in its intrinsic hydrolytic activity and a sustained active state of
KRAS. Mutations in other molecules may also lead to
RAS overstimulation, such as loss-of-function mutations in neurofibromin 1 (
NF1). In most melanomas with altered
NF1, a loss-of-function mutation is found, in which neurofibromin loses its ability to inactivate
RAS and promotes stimulation of the
RAF and its downstream targets, leading to stimulation of the
MAPK pathway and consequent cell proliferation and survival
[21].
Telomerase reverse transcriptase (
TERT) promoter mutations frequently occur in melanoma and, according to The Cancer Genome Atlas (TCGA) data, mainly in the mutated subtypes
BRAF (75% of cases),
RAS (72% of cases), and
NF1 (83% of cases), suggesting a link between
MAPK activation and
TERT expression. The active
MAPK pathway promotes phosphorylation and activation of the ETS1 transcription factor by
ERK (the mutated
TERT promoter bears ETS-binding sites)
[22].
2.2. PI3K-AKT Pathway
The phosphatidylinositol-3-kinases (
PI3Ks) comprise a family of lipid kinases with regulatory roles in many cellular mechanisms, including cell survival and growth, differentiation, proliferation, transcription, and translation. The pathway transduces signals from a variety of growth factors and cytokines and is the major downstream effector of RTKs and G-protein-coupled receptors (
GPCRs) (
Figure 1). Activated
PI3K leads to the formation of phosphatidylinositol-3,4,5-trisphosphate (PIP3) through phosphorylation of phosphatidylinositol-4,5-diphosphate (PIP2) in the plasma membrane. PIP3 is essential for the recruitment of the serine-threonine protein kinase
AKT to the plasma membrane.
AKT is crucial in this signaling pathway, transmitting signals by phosphorylating different downstream effector targets
[23]. Once
AKT is phosphorylated and fully activated, it turns on a major downstream effector of the
PI3K pathway, inhibiting or activating a variety of targets and regulating important cellular processes, such as apoptosis, DNA repair, cell cycle, glucose metabolism, cell growth, motility, invasion, and angiogenesis. The main target of
AKT is the mammalian target of rapamycin (
mTOR), which has a central role in the
PI3K-AKT pathway and cancer disease.
mTOR plays a crucial part in regulating cell growth and proliferation by monitoring nutrient availability, cellular energy, oxygen levels, and mitogenic signals.
PI3K-AKT signaling has negative regulators, to control any persistent and long-term activation. A major regulator of
PI3K-AKT signaling is the tumor suppressor phosphatase and tensin homolog (
PTEN), which antagonizes the
PI3K activity through its intrinsic lipid phosphatase activity, converting PIP3 back to PIP2. Loss of
PTEN results in constitutive activation of
AKT and has been largely associated with tumor development in malignant melanoma. Indeed,
PTEN loss has been shown to be predictive of shorter overall survival (OS)
[24][25].
The
PI3K signaling cascade is upregulated in different types of cancer, including melanoma. More than two-thirds of primary and metastatic melanomas show high levels of phosphorylated
AKT, suggesting that this alteration is an early event in melanoma pathogenesis. Oncogenic events that activate
PI3K-AKT may include mutations or copy number variations in certain components of the pathway.
RAS gene mutations and mutated or amplified expression of RTK may also hyperactivate the
PI3K-AKT pathway
[20]. Mutations in the
mTOR gene are present in approximately 10% of melanomas, and this molecular event leads to shorter survival and worse prognosis
[26].
PI3K-AKT signaling may also be activated in melanoma due to loss of function of the negative regulator
PTEN, which occurs in 10–30% of cutaneous melanomas, leading to constitutive activation of the
PI3K pathway. Interestingly,
PTEN gene alterations are mutually exclusive with
NRAS mutations, and approximately 20% of melanomas with loss of
PTEN function also have
BRAFV600E mutations
[27].
2.3. CDKN2A, Cell Cycle, and Apoptosis Regulation
The
CDKN2A gene encodes two proteins, p16
CDKN2A and p14
CDKN2A, which have a tumor suppressor function. The cyclin proteins bind and activate CDKs, which has catalytic kinase activity. Several cyclin/CDK complexes have been identified that functionally act in different cell cycle phases: in the pre-replicative stage (G1), DNA duplication (S), and promotion of progression through the S phase to mitosis (
Figure 1)
[28]. p16
CDKN2A and p14
CDKN2A proteins have an inhibitory function, interfering with the activity of the cyclin/CDK complexes. p16
CDKN2A inhibits the cyclin D1 (
CCND1)/
CDK4 complex, which, in turn, phosphorylates pRb and allows progression through the G1–S checkpoint. p14
CDKN2A is an antagonist of the mouse double minute 2 homolog (MDM2) protein. This protein degrades p53 and eliminates p53 control of cell growth. The p14
CDKN2A protein inhibits the oncogenic actions of
MDM2 by blocking its actions on p53
[28]. p53 is a transcription factor that functions as a major negative regulator of cell proliferation and survival. Inactivation of the
TP53 gene results in intracellular accumulation of genetic damage, which promotes melanoma development and progression.
TP53 can be inactivated through silencing or mutation, the latter occurring most frequently in high-cumulative solar damage-associated (CSD-associated) melanomas
[29].
Somatic impairment of the
CDKN2A gene in melanoma can occur by genetic deletions, inactivated mutations, or promoter hypermethylation and leads to a decrease of the function of p16
CDKN2A and/or p14
CDKN2A proteins, with consequent loss of cell cycle control. This situation is associated with a higher melanoma invasion potential and metastases
[30].
As mentioned above, mutation of the
CDKN2A gene at the germline level is the most frequent genetic alteration in patients with a strong familial history of melanoma. In addition, variants of the
MC1R gene increase the melanoma risk in
CDKN2A mutation carriers
[31].
The
CCND1 and
CDK4 genes are found to be altered in a minority of melanomas, representing less than 5%, and depend on the melanoma type.
CCND1 gene amplifications affect about 30% of acral melanomas, 11% of lentigo maligna melanomas, and 6% of superficial spreading melanomas.
CDK4 gene amplification is frequently found in acral and mucosal melanomas
[32].
2.4. MITF Pathway
The microphthalmia-associated transcription factor (
MITF) acts as a master regulator of melanocyte development, function, and survival by modulating differentiation and cell cycle progression genes
[33]. It is involved in the differentiation and maintenance of melanocytes and modulates melanocyte differentiation and pigmentation (
Figure 1). In melanomas,
MITF can behave as an oncogene, and in approximately 20% of melanomas, it amplifies and promotes the proliferation of tumor cells. Its amplification correlates with a worse prognosis and a lower OS and ChT resistance
[33].
MITF is activated by the
MAPK and cAMP pathways and regulates the transcription of three major pigmentation enzymes (TYR, TYRP1, and DCT)
[34]. In melanoma,
ERK activity stimulated by
BRAF is associated with
MITF ubiquitin-dependent degradation.
BRAF can modulate intracellular MITF protein through two opposite mechanisms. On the one hand, it can degrade the MITF protein; on the other hand,
BRAF can stimulate transcription factors that increase the expression of the MITF protein. About 10–15% of melanomas harbor the
BRAF mutation along with
MITF amplification, suggesting that additional mechanisms are involved in
ERK-dependent degradation of
MITF.
3. The Integration of Histology and Molecular Diagnostics of Melanoma
Despite recent molecular advances in melanoma characterization, paramount to diagnosis of a melanocytic skin lesion is the integration of several histopathological criteria with the clinical features. In many cases, general morphological criteria for atypia are often the subject of disagreement and inter-observer variability, especially in non-conventional lesions
[35]. The World Health Organization (WHO) recognizes these challenges and incorporate the known molecular pathways in the latest WHO melanocytic tumor classification, introducing the concept of “intermediate” lesions. As stated in a recent review on the topic, this multidimensional classification showed that the view of melanocytic tumors as either benign or malignant might no longer be the proper approach
[36]. Thus, WHO 2018 indicates nine categories/pathways leading to melanoma, each with specific genetic drivers (
Table 1). Furthermore, melanomas can be clustered in three main subtypes, according to the degree of CSD (
Table 1 and
Figure 2)
[37]. The largest group are melanomas associated with low-CSD or superficial spreading melanomas, which often arise on the trunk and proximal areas of the extremities. The most frequent molecular alteration in these melanomas is the
BRAFV600E mutation
[38]. In addition,
TERT promoter mutations and
CDKN2A mutations are also found in the majority of cases.
PTEN and
TP53 are commonly observed in advanced tumors. Lentigo maligna and desmoplastic melanomas are considered tumors associated with high-CSD. These melanomas arise on heavily sun-damaged skin, such as the face or hands, and affect older people. Molecularly, they often have a high mutation load and may harbor
NRAS, BRAF non-V600E, or
NF1 mutations.
TERT promoter mutations and
CDKN2A are also frequently found in these melanomas, and
KIT mutations are found in a subset of cases. Interestingly, the number of mutations increases with the CSD grade (
Figure 2), and desmoplastic melanomas harbor the highest tumor mutation burden. The category of “low to non-UV exposure/CSD” melanomas includes Spitz melanomas, acral melanomas, mucosal melanomas, melanomas developed from congenital nevi and blue nevi, and uveal melanomas. These melanomas rarely harbor
BRAF,
NRAS, or
NF1 mutations (triple wild-type)
[37]. A subset of acral and mucosal melanomas may have
KIT mutations, in addition to gene amplifications and structural rearrangements, most frequently of the
CCND1 gene and
SF3B1. Therefore, genomic studies have subsequently exemplified that acral and mucosal melanomas are biologically distinct from their cutaneous counterparts at sun-exposed sites. Spitz melanomas show a particular oncogenic signaling pathway involving tyrosine kinase or serine-threonine kinase fusions, and melanomas in blue nevus and uveal melanomas are characterized by
GNA11 or
GNAQ mutations
[38].
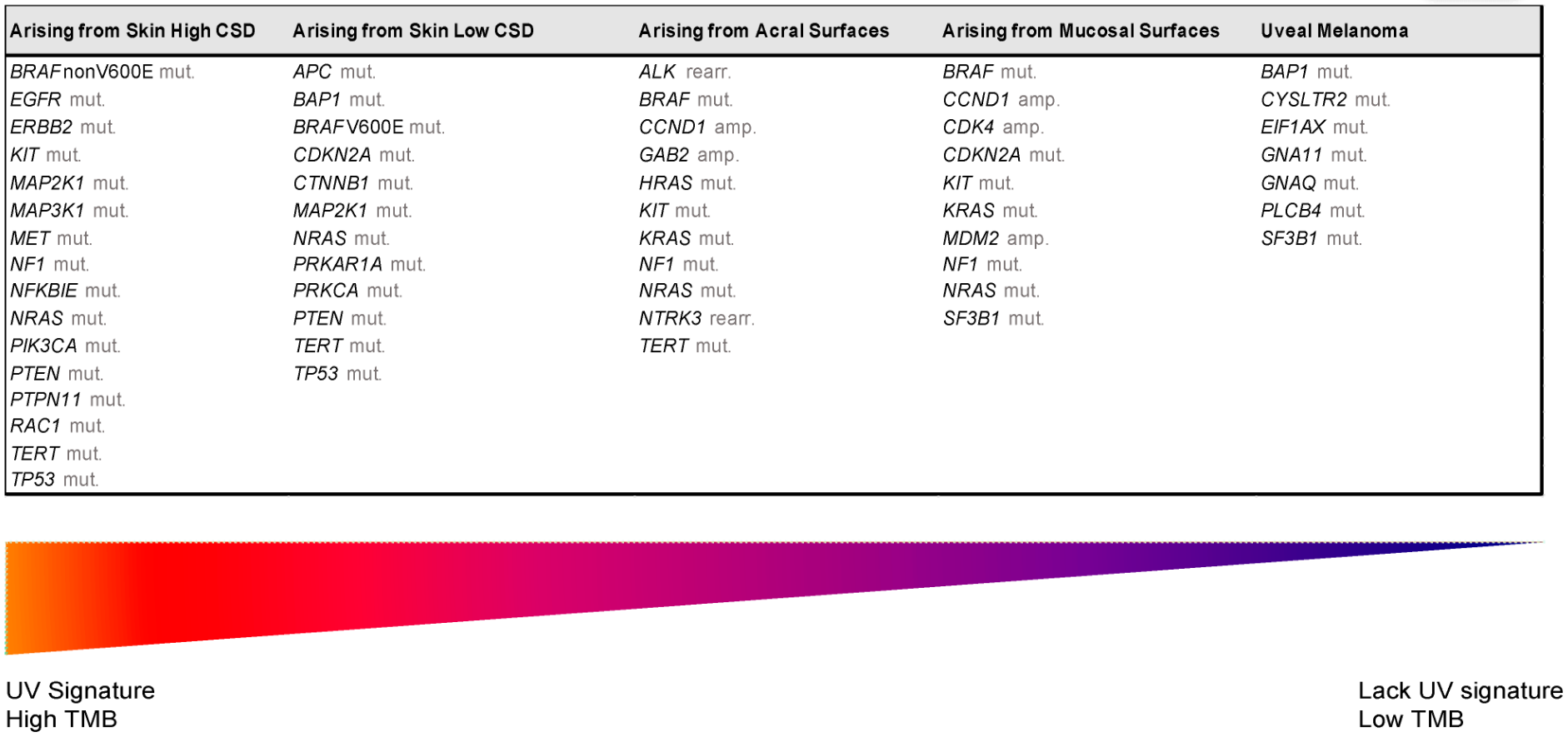
Figure 2. Genomic alterations of melanoma subtypes defined by UV exposure. Abbreviations: amp, amplification; CSD, cumulative sun damage; rearr, rearrangement; TMB, tumor mutational burden; UV, ultraviolet.
Table 1. The classification of melanomas (modified from 2018 World Health Organization Classification).
Abbreviations: amp, amplification; CSD, cumulative sun damage; mut, mutation; rearr, rearrangement.
Certainly, to reduce diagnostic uncertainties and maintain a diagnostic approach based on the WHO 2018 classification, histological assessment should be accompanied by basic immunohistochemistry (IHC) and molecular tests. Recent recommendations of the European Society of Pathology, the European Organization for Research and Treatment of Cancer, and the EURACAN for the diagnosis of intermediate melanocytic proliferations and melanoma variants indicate that most pathology laboratories should perform basic IHC tests, such as: HMB-45; SOX10; MITF, tyrosinase, MART-1; P16; Ki-67/MIB1; BAP1 (BRCA1-associated protein 1); β-catenin; PRAME; and at least one molecular method to detect
BRAF codon 600 and
NRAS mutations
[36]. The most difficult cases that require complementary studies should be analyzed in specialized referral centers, where laboratories can determine a higher grade in a given lesion or the identification of molecular targets that can benefit from targeted therapy.