2. Vascular Occlusions
Canonical thrombi, which form after activation of the cascade of coagulation, are the main cause of vascular occlusions, including arteries, veins, and capillaries. Occluding thrombi hinder blood flow, as they can occupy the entire lumen of vessels. Thromboembolism occurs when a partial thrombus detaches from the vessel walls, floats in the circulation, and eventually fully occludes a distal part of the vessel. Due to its close relationship to inflammation, thrombosis plays a critical role in the pathophysiology of various diseases
[10][11].A number of factors can initiate thrombosis, but the role of NETs in thrombogenesis was reportedly unique
[12].Especially the elucidation of novel roles of extracellular DNA and histones in the bloodstream contributed to the reinterpretation of thrombogenesis. In 2013, the term “immunothrombosis” was coined to describe thrombi initiated by the innate immune response
[13][14].
Fuchs et al. first reported the prothrombotic effect of NETs in 2010
[15]. NETs have been shown to increase thrombosis both directly and indirectly. It remains controversial whether full-size NETs, aggNETs, or the remnants of NETs are the main trigger. While intact aggNETs are thought to act as a scaffold for vascular occlusion, promotion of coagulation by NETs components, including extracellular DNA, via both the intrinsic and extrinsic pathways has also been reported
[16][17]. The mechanisms of NETs-induced thrombogenesis have attracted much attention and have been the subject of numerous investigations, as they are associated with many diseases (
Figure 2). Several biomarkers, including miR-146a
[18], fibrin
[19], plasmin
[20], Sirtuin 3 (Sirt3)
[21], peptidylarginine deiminase type IV (PAD4)
[22], high-mobility group box 1 protein (HMGB1)
[23], and cholesterol crystal (CC)
[24], have been proposed to be involved in NETs-related non-canonical vascular occlusion, which may additionally promote canonical thrombogenesis. Jimenez-Alcazar et al. identified a non-canonical mechanism for vascular occlusion based on the use of targeted mutations in deoxyribonuclease 1 (DNase1) and DNase1l3
[25].
When the dual host protector function of these DNases against the detrimental effects of intravascular NETs is lost, NETs cannot be efficiently removed, and aggregated NETs can occlude vessels.
Figure 2. Schematic representation of aggNETs-driven pathologies. The neutrophils, as a first line of defense, eject NETs when they encounter foreign agents. Single or aggregated NETs might cause, under certain conditions, the obstruction of the flow of body fluids and, consequently, disease. The pathology depends on the site of occlusion; vascular NETs formation is associated with both canonical and noncanonical thrombogenesis in various diseases. The accumulation of aggNETs in the lower respiratory tract has been detected in cystic fibrosis patients. Exocrine gland obstruction has been observed in the pancreas and Meibomian and salivary glands. Large organs like the liver and kidneys can also be affected by the accumulation of NETs. The cartoons were modified from
https://smart.servier.com accessed on 20 August 2021 in compliance with the terms of the Creative Commons Attribution 3.0 Unported License (CC BY 3.0).
2.1. Vascular Occlusions in COVID-19
SARS-CoV-2 (severe acute respiratory syndrome coronavirus 2) continues to be a gobal public health concern. NETs reportedly contribute to acute respiratory distress syndrome (ARDS), and fibrin-based occlusions have been observed in the vasculature of patients with coronavirus disease 19 (COVID-19)
[26][27]. Experimental data demonstrated a positive correlation between the quantity of NETs markers in plasma or serum from patients with COVID-19 and the severity of COVID-19. Increased levels of circulating NETs can cause and exacerbate thrombotic events. In severe COVID-19, intravascular aggregation of NETs causes vasculopathy and can occlude the microvasculature of virtually all organs, leading to organ failure and significant mortality
[27][28].
Indeed, severe COVID-19 is often characterized by vascular occlusions caused by intravascular NETs
[8][29][30]. The lung is the first tissue affected in patients with COVID-19. Patients were found to have higher levels of aggNETs in the lungs, blood, and tracheal aspirate
[31][32]. NETs are capable of directly activating endothelial cells, inducing major changes that include cell death. This promotes endothelial dysfunction, fosters pulmonary edema, and impairs the barrier function at the air/blood interface
[33]. Furthermore, endothelial activation facilitates thrombosis triggered by the accumulation of NETs in the microvasculature
[34]. The fact that classical antithrombotic treatments are hampered in immunothrombosis suggests that NETs are central components of vascular occlusion
[25].
2.2. Coronary Occlusions
Cardiovascular diseases are the most common diseases with the highest mortality rates worldwide
[11]. IIn myocardial infarction, rupture of the atherosclerotic plaque with subsequent thrombogenesis is considered the essential event in arterial occlusions because it jeopardizes epicardial flow. However, the fundamental mechanisms that cause coronary occlusions are not fully understood
[11][16]. Neutrophils have been shown to exacerbate vascular occlusion, and their blood concentration is considered one of the most reliable indicators of acute coronary events. However, their role in coronary thrombosis is relatively understudied compared with monocytes
[35].
The discovery of NETs components in excess amounts not only in plasma and plaques from atherosclerosis patients
[36] but also in atherosclerotic lesions in mice
[37] suggests a pivotal role of NETs in atherosclerosis. This is further supported by the fact that inhibition of DNA decondensation during NET formation, e.g. by inhibitors of PAD4, ameliorates atherosclerosis
[37]. Analysis of thrombus compositions is required to understand the underlying mechanisms of thrombogenesis. A substantial burden of NETs and their critical constituents were detected displaying a heterogeneous morphology in thrombectomy specimens from patients with stent thrombosis
[38] and acute myocardial infarction
[39]. In addition to the high amount of NETs in the thrombi, NETs components such as double-stranded DNA, NE, and MPO were detected in the vicinity of coronary thrombi but not in healthy vessels
[40][41]. The associated viral infections, including COVID-19, have also been detected in the pathogenesis of myocardial infarction. NETs in coronary thrombosis have shown a crucial association with the pathogenesis of ST-elevated myocardial infarction (STEMI)
[42]. Thrombin-activated platelets interact with neutrophils at the site of plaque rupture during acute STEMI, resulting in local NET formation and the release of active tissue factor (TF)
[41]. Significantly elevated NETs-associated TF was found in coronary plasma samples from patients with STEMI
[43].
These findings support the hypothesis that NETs can be targeted to develop new drugs to prevent cardiac thrombosis accompanied by inflammatory conditions like viral infections.
2.3. Cerebral Occlusions
Thromboembolic occlusions in the large cerebral arteries such as the internal carotid (ICA) and/or middle cerebral artery (MCA) cause ischemic strokes, which are the second leading cause of morbidity and mortality worldwide. Treatment involves two strategies: (I) mechanical thrombectomy and (II) intravenous injection of recombinant tissue plasminogen activator (tPA)
[44][45]. More detailed analyses of clots causing ischemic stroke showed that neutrophils and NETs play pivotal roles in the pathogenesis of cerebral occlusions
[44][45]. ]. Indeed, NETs were detected in high concentrations not only in plasma
[46] but also in cerebral thrombi
[47] of stroke patients. In addition, the distribution of NETs in the thrombi from acute ischemic stroke patients was investigated. NETs were detected in 35 of 37 thrombi, especially in fibrin-rich areas
[48]. Thus, new anti-NETs approaches have gained importance for the development of therapies. In this context, treatment with DNase1 and inhibition of PAD4 are considered good candidates
[49].
2.4. Sickle Cell Disease
Sickle cell disease (SCD) is defined as the aberrant production of hemoglobin S (HbS) caused by mutations in the hemoglobin beta chain. The inability to carry sufficient amounts of oxygen results from a single amino acid substitution in the gene encoding the β-globin subunit. The mutations are the hallmark of SCD
[50]. Inflammation and recurrent painful vaso-occlusive crisis (VOC) are commonly seen in the course of SCD. It was previously reported that rigid red blood cells (RBCs) obstruct the microcirculation, and thus cause VOC. The underlying mechanisms of vaso-occlusion have been considered more complicated, and further research is needed for clarification
[50][51]. Erythrocytes are increasingly understood to be a key factor in prolonging the chemokine half-life in the circulation. They serve as dynamic cytokine reservoirs even under healthy conditions
[52]. TIntravascular hemolysis of abnormal hemoglobin was discovered to initiate vascular inflammation due to the release of cytokines and other inflammatory mediators, inducing excessive NET formation during the VOC of SCD
[53][54]. A recent study confirmed the parallel induction of both NETs and inflammatory agents in plasma collected during VOC compared with steady-state conditions
[55]. To provide new therapeutic options, regulation of NETs during VOC is currently under investigation
[56].
3. Airway Occlusions
Human respiratory syncytial viruses (hRSV) are the major viral pathogens causing lower respiratory tract diseases, particularly in neonates and children under five years of age
[57]. In severe RSV infection, the virus exaggerates the activity of neutrophils and eosinophils and causes severe accumulations of neutrophils in the lower airways of the lungs. This causes airway obstruction through the blockage of small airways, which impedes proper breathing and leads to acute morbidity. Excessive formation of NETs is considered to be one of the leading causes for these small airway obstructions (
Figure 2)
[57][58][59].
To develop new therapeutic strategies, the mechanisms of NET formation in the context of hRSV infections have been studied. Funchal et al. showed that the RSV Fusion protein plays a central role in NETs induction via the TLR-4 or ERK/p38 MAPK pathway
[60]. Another comprehensive study postulated that RSV causes NET formation via a canonical ROS-dependent mechanism
[61]. The signal inhibitory receptor on leukocytes (SIRL)-1 and leukocyte-associated immunoglobulin-like receptor (LAIR)-1 have been proposed as potential targets that reduce neutrophil activity and thus regulate airway inflammation upon engagement of these receptors
[62]. Inhaled DNase 1 treatment has been proposed as an alternative therapeutic approach in NETs-induced airway obstruction during severe RSV infection
[63].
4. Occlusions of Exocrine Glands and Ducts
4.1. Pancreatic Duct
The pancreatic duct, also known as the Wirsung duct, connects the pancreas and intestine through the common bile duct. Its primary function is to transport enzymes and bicarbonate, which aid digestion and neutralize the duodenal pH, respectively [64][65]. Occlusion of the pancreatic duct may cause pancreatitis. The occlusions of the ducts are directly proportional to the severity of the pancreatitis and depend on the duration of the disease [64][65].
NETs have been reported to directly induce trypsin activation, inflammation, and tissue damage in severe acute pancreatitis induced by retrograde taurocholate infusion [66]. However, the direct cause of obstruction is not always found in human acute pancreatitis. Under physiological conditions, neutrophils are present in small amounts in the pancreas and enter the bicarbonate-rich pancreatic fluid and spontaneously form NETs [67]. In the case of severe inflammatory situations, the resulting neutrophilia can produce excessive amounts of NETs in the pancreas. This results in large and sticky aggregates prone to occlude pancreatic ducts. Leppkes et al. reported that sole neutrophilia in IL17 transgenic mice was the main driving force for the development of pancreatitis. In this case, excessive NETs formation was immediately induced by a high bicarbonate concentration in the pancreatic juice. Formed NETs tend to aggregate and occlude the ducts triggering focal acute pancreatic [68]. The reduced production and sizes of NETs and aggNETs in the pancreatic ducts of PAD4-KO mice prevented the development of focal pancreatitis [69][70].
4.2. Meibomian Gland
The Meibomian gland (MG) secretes a biological fluid (meibum) containing a significant amount and variety of lipids. Meibum is crucially important for the maintenance of a healthy ocular surface. Meibomian gland dysfunction (MGD) and the disruption of meibum homeostasis change the lipid content of the tear fluid
[71][72]. The lack of lipids in the tear film promotes hyper-evaporation and tear hyperosmolarity.
As neutrophils are the first cells to be recruited to the foci of inflammation, NETs have been discussed to drive the pathogenesis of MGD-related diseases, including dry eye disease (DED). The increased abundance of NETs in the tears and on the ocular surfaces of the patients with MGD and the positive correlation between NETs amounts and disease severity has reinforced this suspicion
[73]. Although aggNETs have been known for their ability to resolve inflammation on the ocular surface
[74], a recent study demonstrated that NETs are also implicated in MG terminal duct occlusions precipitating the inflammation on the ocular surface
[9]. This work has provided vital new evidence to propose novel avenues in the treatment of MG occlusion-related disorders. In addition to the currently available medical treatments, such as antibiotics and nonsteroidal and steroidal anti-inflammatory drugs, NETs formation inhibitors should be considered in the therapeutic arsenal of MGD
[75].
4.3. Periodontitis (Periodontal Crevicular Occlusions)
The gingival crevice, also called the gingival sulcus, is defined as a narrow V-shaped space between the inner aspect of the free gingival epithelium and the surrounding enamel of a tooth. Normally, its depth range is 1–3 mm. The gingival epithelium continuously produces gingival crevicular fluid (GCF) that is finally transferred into the oral cavity. It has been known for many years that the production of GCF and its composition change during inflammatory diseases like periodontitis. Therefore, GCF has been extensively studied as a diagnostic tool [76][77]. Under physiological conditions, the minimal amount of GCF present in the gingival crevice flows into the oral cavity, making the crevice a kind of duct. Upon inflammation, this fluid transforms into a purulent exudate containing large amounts of NETs and neutrophils expressing CD177 [78][79]. The exudate is extremely viscous due to the excessive amount of aggNETs. Vitkov and colleagues put forward the view of NETs-driven obstruction of the periodontal crevice. The authors speculated that the formation of a periodontal abscess might result from NETs-induced cervical obstructions [6] supported by the overproduction of NETs and the impaired clearance of NETs remnants [80]. This hypothesis has not yet been proven experimentally. However, the crucial roles of NETs in the initiation and progression of inflammatory periodontal diseases are surely worth investigating.
4.4. Gallstones
Obstruction of the biliary system, a common, serious, and painful condition, is one of the leading causes of hospitalization with significant morbidity and mortality. The formation of gallstones in the gallbladder or ducts, also referred to as cholelithiasis, are the most prevalent etiological event for biliary obstruction that results in biliary stasis. However, gallstone-caused biliary obstructions generate a high socioeconomic burden due to their high incidence, especially in developed countries [81][82]. Until recently, it has been proposed that gallstones simply form due to the supersaturation of cholesterol crystals. The contents of human gallstones were eventually investigated for NETs, as cholelithiasis is an occlusive condition [83]. After observing extracellular DNA and neutrophil elastase in gall sludge and gallstones, it was established that an intact NETs formation capacity is necessary to form gallstones in a murine model of cholelithiasis. Basically, NETs were crucial in the initiation and progression of gallstone formation by promoting the aggregation of biliary cholesterol and calcium crystals. Furthermore, a positive correlation between the neutrophil/lymphocyte ratio and the severity of gallstone-induced pancreatitis has been reported in clinical settings [84]. However, formal clinical trials employing NETs formation inhibition in patients with recurrent cholelithiasis are still needed [85].
4.5. Sialoliths
Sialoliths, also known as salivary stones, are the most prevalent obstructive disease of the salivary glands, especially for middle-aged patients. Stone formation is mostly seen in the submandibular gland, with an incidence of more than 80 percent. The parotid gland follows it with 13 percent and by sublingual and minor salivary glands at very low rates. The etiology of sialolith formation was initially considered a multifactorial interaction of calcium salts, organic and inorganic molecules, pH, and bacteria [86][87]. In two conflicting reports, inorganic materials [88] and organic components [89] have claimed to be the main components of sialoliths. However, since the role of inflammation in sialoliths formation [90] and the presence of bacterial residues, including bacterial DNA and biofilms in their structures, has been established [91], NETs have gained attention as a possible etiological factor. Furthermore, since bacterial biofilm structures at the core of sialoliths have been established, biofilms have been hypothesized to be an initial step in the formation of sialoliths [92]. Recently, neutrophil recruitment and NETs formation in the salivary system were suggested to initiate sialolithiasis. Indeed, the demonstration of NE activity associated with a high prevalence of extracellular DNA points to NETs as nidi for the formation of sialoliths [93]. The high content of bicarbonate in saliva strengthens this hypothesis as it facilitates the formation of NETs [67]. The interaction between neutrophils and the precipitated particulate matter is discussed to cause the salivary stone to grow. These results offer an alternative perspective for the “until now” proposed mechanisms of sialolithogenesis [94][95].
5. Therapeutic Approaches to Prevent NETs-Driven Occlusions
Additionally to their protective effects against pathogens, NETs contribute to the regulation of innate and adaptive immunity. In the case of persistent neutrophil activation, the NETs form aggregates that contribute to the resolution of inflammation, reflecting the advantageous side of NETs formation. Depending on where aggNETs are formed (e.g., vessels or ducts), these large and sticky aggregates might cause various kinds of obstructions. These occlusions drive the pathophysiology and increase the severity of many diseases, including COVID-19, chole- and sialolithiasis, dry eye disease, and probably many others. Therefore, it is critical to developing new effective therapeutic strategies directed to control either the accumulation of NETs or improving their clearance at specific anatomic locations
[26][96]. NETs-inducing agents are diverse, and the formation mechanisms of NETs vary depending on these triggering agents.
Consequently, the mechanism of NETs formation has to be explored for every single condition, and then, therapeutic strategies have to be developed in a condition-specific manner. NE, MPO, PAD4, and extracellular DNA are promising targets for suppressing excessive NETs formation
[11][28] and are, therefore, currently the focus of drug development (
Figure 3). Among these, the inhibition of PAD4 and the treatment with DNase1 are the most promising candidates for NETs-driven occlusions
[80]. Therefore, researchers will explore these two candidates together with others in more detail in the following paragraphs.
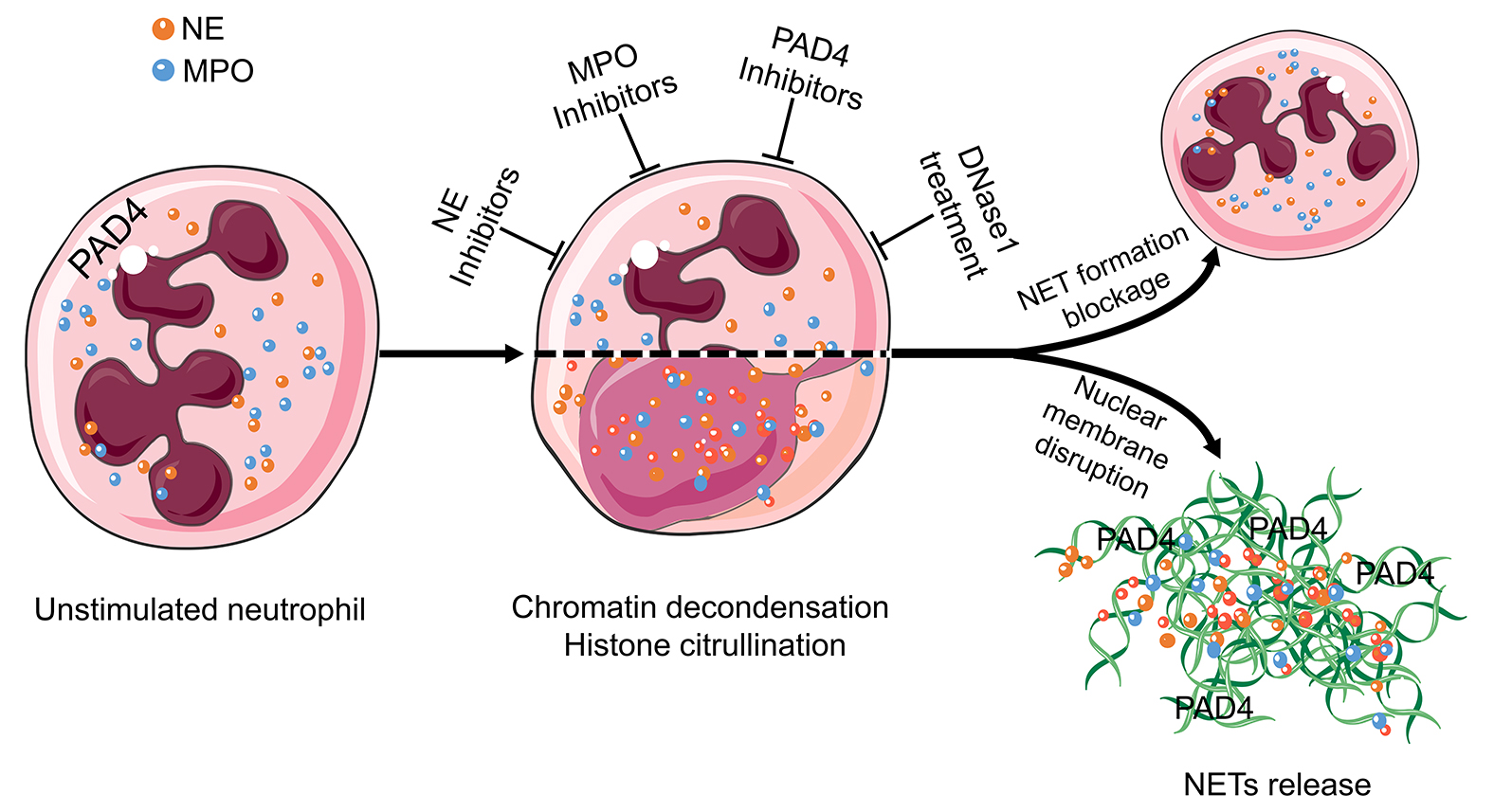
Figure 3 Targets in the treatment of aggNETs-driven occlusive pathologies. NE, MPO, PAD4, and extracellular DNA are promising targets for the suppression of excessive NETs formation. Enzymatic inhibitors of PAD4 and MPO have demonstrated its effectivity in preclinical settings of cholelithiasis, Meibomian gland dysfunction, and vasculitis. DNase treatment is effective at preventing death in severe sepsis and improving the symptoms in cystic fibrosis, bronchiolitis, and dry eye disease. The cartoons were modified from https://smart.servier.com accessed on 20 August 2021 in compliance with the terms of the Creative Commons Attribution 3.0 Unported License (CC BY 3.0). Abbreviations: MPO, myeloperoxidase; NE, neutrophil elastase; NET, neutrophil extracellular traps; and PAD4, peptidylarginine deiminase type IV.
5.1. Inhibition of PAD4 Reduces Formation and Size of NETs
Peptidylarginine deiminases (PADI) are a calcium-dependent enzyme family responsible for post-transcriptional deamination/citrullination. In this process, the positively charged arginine residues are converted into uncharged citrulline residues. The PADI family consists of five members. PAD4 is unique among them. It plays a role in the formation of NETs by ensuring the decondensation of chromatin [97]. This makes PAD4 a possible therapeutic target for the treatment of occlusive NETs-related diseases. The increased activity of PAD4 and the therapeutic potential of its inhibition have already been reported in preclinical settings for the formation of thrombosis [22], acute pancreatitis [70], cholelithiasis [83], meibomian gland disfunction [9], lung injury [98], and sickle cell disease [56].
5.2. Deoxyribonucleases Dismantle NETs
DNases have been studied for therapeutic purposes in obstructive conditions. DNase1 cleaves DNA by breaking its phosphodiester bonds. This disrupts the structural integrity of NETs and reduces the sizes and amounts of aggNETs [99]. It has been shown that disrupting NETs with DNase1 not only prevents vascular occlusion [25] but also recanalizes the already occluded vessel [100]. Similar results have been obtained in crystal clots-driven arterial occlusion [24]. The improvement of ventilation after the inhalation of DNase1 in RSV acute bronchiolitis has also been demonstrated [60]. DNase1 is already approved as an inhalant to reduce the viscosity of the mucus in the lungs of patients with cystic fibrosis [63].
5.3. Inhibitors of Myeloperoxidase Reduce the Early Phases of NET Formation
One of the most important elements of NETs, especially in the early phases, is MPO. When neutrophils encounter danger signals, MPO enters the nucleus and drives chromatin decondensation, a crucial step of NETs formation [101]. Inhibitors of MPO such as PF-1355 have been tried as NETs formation blockers in small vessel vasculitis. In this context, PF-1355 prevented excessive NETs formation and reduced leukocyte infiltration [102], making it a good candidate for further studies trying to interfere with NETs formation in occlusions. Furthermore, natural surfactants also have the ability to inhibit NETs formation in vitro [103]. Therefore, natural surfactants bear the potential to be used as therapeutical agents in occlusive conditions.
6. Conclusions
Neutrophils and NETs participate in the initiation, pathogenesis, and resolution phases of several inflammatory conditions. Considerable collateral damage is expected in most of the cases where neutrophils are involved. Therefore, robust regulatory mechanisms like apoptosis and NETs formation have evolved to endow neutrophils with the ability to drive the amelioration of the initial inflammation. The clearance of apoptotic neutrophils by professional phagocytes triggers potent anti-inflammatory and regenerative responses [104][105]. NETs formation and aggregation actively limit the spreading of pathogens and inflammatory mediators [7][106]. Unfortunately, those anatomical locations carrying fluids or air through the body are prone to be occluded by NETs generating heterogeneous pathologies. The study of the prevention or dissolution of such clogs will provide new therapeutic opportunities for old prevalent diseases.