2. Chemical Structure and Toxic Effects of Mycotoxins
Since 1960, more than 400 forms of mycotoxins have been identified and reported. The most common mycotoxins in food include aflatoxins (AFs), trichothecenes, ochra-toxins, zearalenone (ZEA), fumonisins, and patulin, which are highly toxic
[20]. These notable contaminants can be produced by various toxigenic fungi due to improper harvesting, storage or transport conditions
[21]. Most of them have complex chemical structures containing specific groups that confer the toxic effects. Basic information of the main mycotoxins including chemical structure, biological effect and producing fungi is summarized in
Table 1 [21][22][23][24][25].
Table 1. Chemical structure, toxic groups, biological effects of main mycotoxins and producing fungi.
Mycotoxins |
Chemical Structure |
Main Toxic Groups |
Main Degradation Products |
Organ/System Affected |
Main Clinical Signs |
Producing Fungi |
Aflatoxins (B1, B2, G1, G2) |
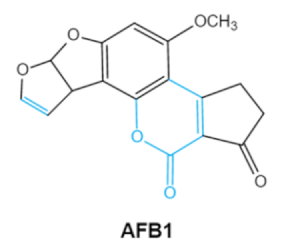
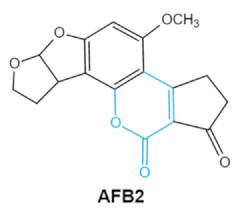
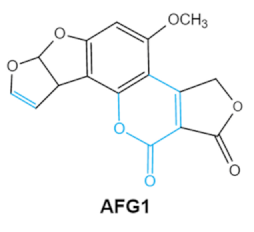
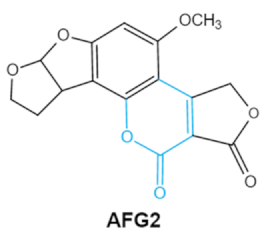 |
Lactone ring Double bond in difuran ring moiety |
AFB1-8,9dihydrodiol, AFB1-8,9-epoxide, dihydrohydroxyaflatoxin B1, |
Liver, kidney, immune system |
Hepatitis, carcinogenic, abdominal pain, vomiting, increased susceptibility to disease, immunosuppressive and carcinogenic effects |
Aspergillus. Flavus A. Parasiticus A. nomius |
Zearalenones (ZEA) |
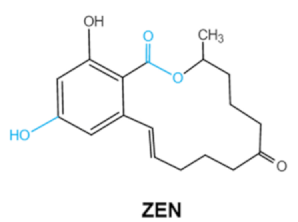 |
Lactone ring C-4 hydroxyl group |
α-/β-zearalenol, α-/β-zearalanol,zearalenone-4-sulfate, 1-(3,5-dihydroxyphenyl)-6′-hydroxy-l’-undecen-l0′-one, (5S)-5-({2,4-dihydroxy-6-[(1E)-5-hydroxypent-1-en-1-yl]benzoyl}oxy)hexanoic acid |
Reproductive tract, mainly female |
Hyperestrogenism, Reproductive disorders |
Fusarium graminearum(F. roseum) F. culmorum F. equiseti F. cerealis F. verticillioides F. incarnatum |
Ochratoxins (A,B,C) (OTs) |
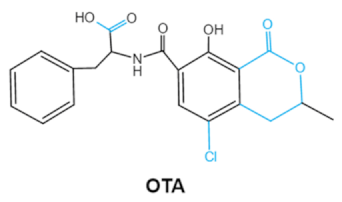
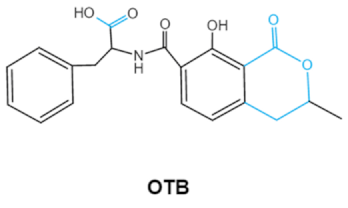
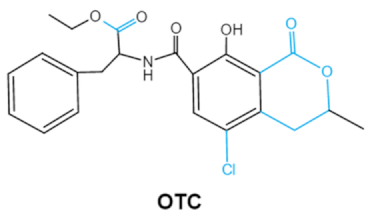 |
Isocoumarin moiety Carboxyl group of the phenylalanine moietyCl group |
L-βphenylalanine, OTα |
Liver, kidney, immune system, inhibit RNA, DNA and protein synthesis in kidney |
Nephritis, enlargement of kidney and hepatitis |
A. ochraceus A. carbonarius A. niger P. verrucosum P. nordicum |
Fumonisins FBs (B1, B2) |
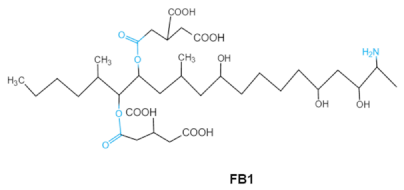
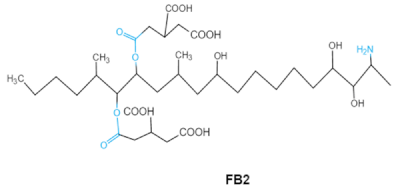 |
Two tricarballylic acid side chains Free amino group |
2-oxo-12,16-dimethyl-3,5,10,14,15-icosanepentol hemiketal, NacetylAP1, |
Lungs and heart (pig), central nervous system (horse), liver, immune system |
Porcine pulmonary edema (PPE), equine leukoencephalomalacia |
Fusarium section Liseola |
Trichothecenes (DON, T-2, HT-2) TCNs |
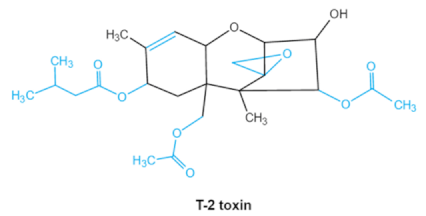
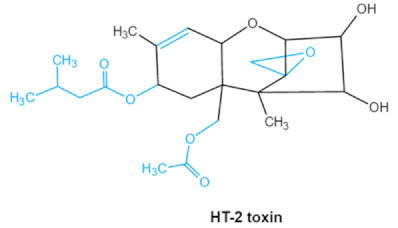
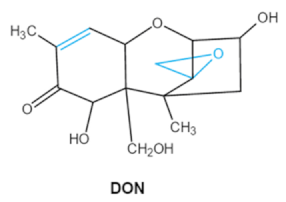 |
Epoxide group Acylated side groups C9-10 double bond |
HT-2 toxin, T-2 triol, T-2 tetraol, de-epoxy T-2 tetraol, 3α,7α,15α-triacetoxy-deoxynivalenol, de-epoxy deoxynivalenol, 3-acetyldeoxynivalenol, diaacetoxydeoxynivalenol. Epoxymonoacetoxyscirpenol, de-epoxyscirpentrio |
Central nervous system, gastrointestinal tract, liver, immune system |
Anorexia, vomiting, abdominal pains, cardiovascular dysfunction |
F. acuminatum F. sporotrichioides F. langsethiae Fusariumgraminearum, F. culmorum F. cerealis F. culmorum F. graminearum F. sporotrichioides F. poae |
Patulin PAT |
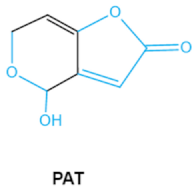 |
Furan, pyran or lactone ring Hemiacetal |
Ascladiol, hydroascladiol, desoxypatulinic acid, 3-keto-5-hydroxypentanal, glyoxylic acid |
Gut epithelium, liver, kidney, immune system |
Oral and epithelial lesion, loss of appetite Nausea, vomitin, gastric ulcers |
Penicillim expansum Bysochlamis nívea Aspergillus clavatus P. griseofulvum |
2.1. Aflatoxins (AFs)
Aflatoxin (AFs) is the most widely studied mycotoxin encountered in agricultural commodities. They are mainly produced by
Aspergillus species,
Aspergillus flavus, A. parasiticus and A. nomius. AFs shave been reported to induce hepatic toxicity, nephron toxicity and immune suppression in humans
[26]. Of the AFs, AFB1 is extremely hazardous and has been considered as a Group I of naturally occurring carcinogens
[27]. AFs are furanocoumarins associated with a bisdihydrodifuran or tetrahydrobisfuran united to a coumarin, replaced by a cyclopentanone or a lactone
[28]. Lactone ring and the double bond in the terminal furan ring of AFs are responsible for the toxicity and carcinogenic activity
[23][29].
2.2. Trichothecenes
As one of the major classes of mycotoxins, trichothecenes (TCNs) cause a significant economic impact on the food chain each year. TCNs are formed in nature by some filamentous fungi such as
Aspergillus, Penicillium, Fusarium, Spicellum and
Stachybotrys, among them
Fusarium is the most common toxin source in cereals, vegetables, and feedstuff
[30][31]. The consumption of TCNs-contaminated food may affect the gastrointestinal tract, kidney, liver, and immune system, and cause health issues such as anorexia, vomiting, abdominal pains and cardiovascular dysfunction
[32]. TCNs are tetracyclic sesquiterpenoid epoxides and form a family of over 200 toxins with an atricyclic 12,13-epoxytrichothec-9ene (EPT) core structure in common
[33]. According to their structure, toxins in this family are classified into four groups (types A, B, C, and D) based on the substitution pattern of EPT, among them, type A and type B are of special interest because of their high toxicity
[34]. Representative type ATCNs includes T-2 toxin (T-2), HT-2 toxin (HT-2) and neosolaniol (NEO), while type BTCNs were represented by deoxynivalenol (DON) and its acetyl derivatives
[35]. The 12, 13-epoxide ring, 9–10 double bond and acylated side groups in trichothecenes are essential for imparting the toxic effects in inhibiting the synthesis of protein, RNA and DNA
[36][37]. Generally, the complete detoxification of TCNs is a challenge due to their stable and complex structure
[38].
2.3. Ochratoxins
Ochratoxins (OTs) belong to a family of mycotoxins commonly found in various food and feed mainly produced by
Penicillium and
Aspergillus species, including
P. verrucosum, P. nordicum, A. ochraceus, A. carbonarius and
A. niger [39]. They comprise a group of coumarin derivatives, including ochratoxin A (OTA), ochratoxin B (OTB) and ochratoxin C (OTC), of which OTA is the most prevalent and toxic compound
[40]. OTA is of neurotoxicity, carcinogenesis, immunotoxicity and hepatotoxicity, which poses a serious threatens to both human and animal health.
[41]. Isocoumarin moiety is the key toxic group of OTA, and the carboxyl group of the phenylalanine moiety as well as the Cl group contribute to its toxicity
[25].
2.4. Zearalenone
Zearalenone (ZEA) is a kind of nonsteroidal mycotoxin that contaminates a variety of cereals
[42]. ZEA is a secondary metabolite that is synthesized by several species of mold fungi belonging to the
Fusarium family that colonizes plants grown in the temperate climate zone such as corn, maize and other grain crops
[43]. ZEA belongs to xenoestrogens that have a chemical structure similar to natural estrogens. Therefore, tZEA can bind to estrogen receptor sites, causing hormonal imbalance that may lead to reproductive diseasesin human and livestocks
[44]. Lactone group in the chemical structure of ZEA is critical to the toxicity of ZEA. It has been reported that the toxic effects of ZEA can be significantly reduced by the cleavage of the lactone ring
[45].
2.5. Fumonisins
Fumonisins (FBs), polyketide-derived mycotoxins, are mainly produced by
Fusarium mold species that commonly infect corn and other agricultural products
[46]. Based on the structural difference, four main groups of fumonisin analogs have been characterized, and among them, FB1 is the most prevalent and toxic
[47]. FBs have a chemical structure similar to sphingolipid long-chain bases, such as sphinganine (Sa) and sphingosine (So) which are intermediate compounds in the sphingolipid metabolism, which can inhibit ceramide synthase and block the biosynthesis of complex sphingolipids, resulting in a wide spectrum of pathological effects in humans and animals
[48]. The tricarballylic acid (TCA) side groups and the free amino group play important roles in FBs toxicity mechanism. It has been found that the removal of these groups can significantly reduce both phytotoxicity and mammalian cytotoxicity
[49][50].
2.6. Patulin
Patulin (PAT) is a tetraketide mycotoxin most commonly found in apples and apple-derived products
[51]. It is produced by a variety of filamentous fungi including
Penicillium, Aspergillus and
Byssochlamys [52]. Acute PAT exposure damages gut epithelium, liver, kidney, and immune system, causing symptoms such as nausea, vomiting and intestinal hemorrhages
[53]. PAT has also been shown to affect the activities of thiol-containing enzymes that play important roles in glycolysis, since this mycotoxin can easily form covalent sulfhydryl-containing compounds, which is the main mechanism associated with PAT toxicity
[54]. The destruction of lactone, furan or the pyran ring and hemiacetal in the structure of PAT may be an indicator of toxicity reduction
[8].
3. Mycotoxin Removal by Probiotics
3.1. Fast Glance to Probiotic Properties
Probiotics, defined as ‘‘live microorganisms which when administered in adequate amounts confer a health benefit on the host’’ by the World Health Organization (WHO), have been incorporated into various kinds of products such as foods, drugs, and dietary supplements
[55]. Beyond their basic nutritional value, probiotics provide numerous benefits such as the alleviation of lactose intolerance, maintaining balance of the digestive tract and modulation of inflammatory responses
[56]. The most extensively studied and widely used probiotics include
Lactobacillus,
Bifidobacterium, yeast
Saccharomyces cerevisiae as well as some
Bacillus species
[57]. Gaggìa et al. (2010) summarized the species of probiotics and their health-promoting characteristics based on extensive studies and an internet search of commercial products
[58]. These nonpathogenic, nontoxigenic, and fermentative microorganisms are quite commonly added to “functional foods” and growth supplements for human health. The application of probiotics is also prevalent in the aquaculture field, acting as alternatives for antibiotics or disinfectants to improve water quality
[59]. Moreover, specific strains of probiotic bacteria were indicated to be effective in removing contaminants such as heavy metals, pesticides as well as mycotoxins, which might act as promising bio-agents for food safety
[60][61][62].
3.2. Mycotoxins Detoxification by Probiotics and the Related Mechanism
The biological detoxification of mycotoxins by probiotic bacteria was reviewed years ago
[28][55][59]. Probiotics, particularly lactic acid bacteria (LAB) and yeasts such as
Saccharomyces genus, can remove mycotoxins primarily through two mechanisms, surface adsorption or biodegradation (
Figure 1). Toxins removal by surface adsorption of microorganisms is a fast and reversible process that does not cause any chemical changes in the substrate. In contrast, biodegradation is permanent but results in undesirable metabolites.
3.2.1. Cell-Binding of Mycotoxins
In the last three decades, numerous studies have been developed regarding toxin binding by the cell-surface of probiotics such as
Lactobacillus rhamnosus, Lactobacillus amylovorus, Lactobacillus plantarum, Lactobacillus pentosus [63][64][65][66]. Most of studies in vitro have been carried out using viable cells or heat-treated cells with aqueous toxic standards. The binding capacity of mycotoxins was strain-dependent, and depends on the natural structure of the toxin as well as physicochemical conditions. In vivo studies in animal trials and human clinical trials investigating the real effect of probiotics binding capacity as antidote for mycotoxin detoxification have been reviewed
[67]. Although certain probiotic strains have shown a positive role in the restoration of mycotoxins damage, studies concerning the fate of the ingested mycotoxins and probiotic-mycotoxin still need to be supplemented.
The structural components of the probiotic cell wall play a pivotal role in the binding of toxins by microorganisms. LAB has the typical cell wall structure of Gram-positive bacteria, which consists of thick and multilayered peptidoglycan (PG) sacculi decorated with other glycopolymers including teichoic acids (TAs), polysaccharides (PSs) and proteinatious Slayer
[68]. The binding efficiency of different LAB species seems most closely related to the amino acid sequence of peptide bridges of the PG
[69]. The cell wall of LAB contains plenty of negatively charged functional groups that might facilitate the binding capacity due to the presence of S-layer proteins
[60]. The literature available has limited information on the role of teichoic acids and polysaccharide involved in the toxins binding mechanism, experimental studies of enzymatic degradation showed that deficiency of these components in cell wall leads to less toxin accumulation
[70][71]. The cell wall of yeasts such as
Saccharomyces genus is mainly composed of an inner layer with β-glucans and chitin, and outer layer with heavily glycosylated mannoproteins. Some studies suggest that the cell wall thickness depends on β-D-glucans reticular organization and that β-(1, 3)-D-glucans contents play a major role in the toxin adsorption efficacy in
S. cerevisiae [72][73]. Thus, elucidating the differences between cell wall components of probiotics species might provide a potential strategy to select strains to act as the mycotoxin binder.
3.2.2. Biodegradation
In contrast to cell-binding methods, research on the biodegradation of mycotoxins by probiotics is very limited. The available literatures about mycotoxins degradation by probiotics over the last decade was summarized in
Table 2. Harkai et al. (2016) successfully selected several
Streptomyces strains which have shown a strong capability to degrade AFB1 and ZON mycotoxin with eliminated genotoxicity
[74]. Rao et al. (2017) reported a well-known probiotic bacteria
Bacillus licheniformis isolate CFR1, which can degrade aflatoxin B1 in liquid culture media with a reduction rate of 94.7%. In the same study, it was found that AFB1 could be metabolized to degradation products using a cell-free supernatant, and that treatments such as heating, proteinase K and SDS lead to the complete loss of degradation activity, indicating that the extracellular proteins or enzymes may be involved in the degradation process
[75]. The application of biological detoxification in food fermentation has enormous practical significance. Juodeikiene et al. (2018) treated wheat grains with three P. pen-tosaceus strains, and the results showed a decrease in mycotoxins (DON, T-2 and HT-2 toxins) in malting wheat grains. The strains would serve as candidates for reducing mycotoxins in corresponding raw materials for beverages and certain baked-goods production.
[76]. During the beer-brewing process, some stable mycotoxins in contaminated cereal-derived raw materials may survive and enter the final products, thereby it is important to screen brewers’ yeast with the ability to alleviate toxicity. In the study conducted by Nathanail et al. (2016), the lager yeast
Saccharomyces pastorianus A15 may enzymatically transform
fusarium trichothecenes to less toxic form in the fermentations of brewer’s wort, with reduction rates of 15% for DON, 17% for DON3Glc, 34% for HT-2 and 31% for T2
[77]. This strain might be an excellent candidate for mycotoxicosis control during food processing. It must be addressed that the biodegradation method may bring potential risks. Most of the studies did not look into the possible toxicity of by-products created during the decomposition process. For biotechnological utilization, applicable methods must be developed to monitor the potential hazardous metabolites and biological effects in mycotoxin biodegradation.
Table 2. Degradation of mycotoxins by probiotic bacterial strains reported in the last decade.
Mycotoxins |
Microorganism |
Reduction Rate (%) |
Toxin Level |
Degradation Condition |
Reference |
AFB1 |
Streptomyces cacaoi subsp. Asoensis K234 |
88.34 ± 15.62 |
1 μg mL−1 |
5 days, 28 °C,170 rpm liquid LB medium |
Harkai et al. (2016) |
Streptomyces luteogriseus K144 |
79.93 |
1 μg mL−1 |
5 days, 28 °C,170 rpm liquid LB medium |
Bacillus licheniformis CFR1 |
94.73 ± 1.09 |
500 ppb |
liquid nutrient broth (NB) at 37 °C, 72 h |
Rao et al. (2017) |
T-2 |
P. pentosaceus KTU05-10 |
78.0 |
12.8–19.5 μg kg−1 |
malting wheat grains with bacterial suspension at 18 °C for 30 min |
Juodeikiene et al. (2018) |
Saccharomyces pastorianus A15 |
31.0 |
5000 µg L−1 |
15 °C, 120 rpm, four days 11.5° Plato wort |
Nathanail et al. (2016) |
HT-2 |
P. pentosaceus KTU05-10 |
79.0 |
258–819 μg L−1 |
malting wheat grains with bacterial suspension at 18 °C for 30 min |
Juodeikiene et al. (2018) |
ZEA |
P. acidilactici |
38.0 |
19.5–873.7 μg L−1 |
malting wheat grains with bacterial suspension at 18 °C for 30 min |
Juodeikiene et al. (2018) |
Streptomyces rimosus (K145, K189) |
100.0 |
1 μg mL−1 |
5 days, 28 °C,170 rpm liquid LB medium |
Harkai et al. (2016) |
DON |
P. pentosaceus KTU05-10 |
47.0 |
3370–6930 μg kg−1 |
malting wheat grains with bacterial suspension at 18 °C for 30 min |
Juodeikiene et al. (2018) |
Saccharomyces pastorianus A15 |
15.0 |
400 µg L−1 |
11.5° Plato wort, 15 °C, 120 rpm for 4 days |
Nathanail et al. (2016) |