Citrus fruits (genus
Citrus in family Rutaceae), comprising oranges, grapefruit, mandarins, limes and lemons, are among the most widespread fruit crop worldwide, highly enriched with components beneficial to human health. The great economic value of the citrus fruits market is also based on properties that largely rely on factors affecting both external and internal quality. While no bacterial postharvest diseases of commercial importance have been reported, citrus fruits are highly susceptible to fungi infection
[1]. Among them, the green mould and blue mould, caused by
Penicillium digitatum and
Penicillium italicum, respectively, represent the two most important diseases in all citrus production during fruits postharvest handling procedures
[2][3]. In particular, the postharvest green mould is the main factor affecting citrus fruit decay, leading to huge economic losses worldwide every year and accounting for up to 90% of the total citrus postharvest losses, especially in subtropical climates
[3].
Synthetic fungicides played a key role in crop protection for the control of the citrus postharvest green mould. However, fungicide resistance represents a critical issue worldwide, with still limited approaches for effective disease management
[4]. Thus, developing efficient alternative approaches for counteracting postharvest diseases of citrus fruits caused by
Penicillium species has become an active field of research. Several promising biological control strategies, including the use of antagonistic microorganisms, the application of naturally-derived bioactive compounds and the induction of natural resistance, have been proposed as potential alternatives to synthetic fungicides for the control of citrus diseases
[1].
These approaches rely on naturally occurring agents such as several secreted proteins from fungi endowed with antiviral, antibacterial, antifungal, and insecticidal activities such as the antifungal protein (AFP) and the ribotoxin α-sarcin from
Aspergillus giganteus [5][6][7][8]. Recently, it was reported that α-sarcin, traditionally considered toxic only to animal cells, displays a strong antifungal activity against
Penicillium digitatum for its ability to enter into the cytosol and inactivate the ribosomes, thus killing cells and arresting fungus growth
[9]. Ribotoxins such as α-sarcin are rRNA endonucleases (EC 4.6.1.23) that catalyse the cleavage of the phosphodiester bond on the 3′ side of the G4325 residue from the rat large rRNA. This nucleotide is located in the Sarcin Ricin Loop (SRL) that is involved in the binding of the elongation factor to the ribosome
[9]. In addition, several plant-derived proteins such as the ribosome-inactivating proteins (RIPs) have been extensively studied for their antiviral, antifungal and insecticidal activity mediated by the inhibition of protein synthesis
[10][11]. RIPs belong to a class of enzymes (EC 3.2.2.22) that exhibits rRNA N-glycosylase activity. This activity prevents protein synthesis by causing the release of a specific adenine residue in the SRL of the large rRNA. A marked antifungal activity against the green mould
Penicillium digitatum has been described for the apoplastic protein beetin 27 (BE27), a RIP isolated from sugar beet (
Beta vulgaris L.) leaves. BE27 is able to enter into the cytosol and kill cells, thus arresting the growth of the fungus at a concentration much lower than that present in the apoplast
[12].
2. Deciphering Molecular Determinants Underlying Penicillium digitatum’s Response to Biological and Chemical Antifungal Agents by Tandem Mass Tag (TMT)-Based High-Resolution LC-MS/MS
Over the past decade, the technological advances in mass spectrometry (MS)-based proteomics, together with the availability of
P. digitatum and other fungi genome sequence information, enormously increased the potential to characterize the molecular changes occurring within pathogenic fungi in response to treatments with novel biofungicides. Recently, a label-free quantitative proteomic analysis was performed on
Fusarium oxysporum f. sp.
cucumerinum mycelia following treatment with canthin-6-one, an alkaloid compound extracted from
Ailanthus altissima, with the aim of investigating the molecular mechanisms underlying the antifungal properties of this molecule
[13]. A similar approach was applied to decipher the effects of the antifungal peptide ETD151, an analogue of the antifungal insect defensin heliomicin, on the phytopathogenic fungus
Botrytis cinerea [14]. In addition, an iTRAQ-based LC-MS/MS analysis was used to evaluate the proteomic profiling of
B. cinerea in response to wuyiencin, produced by
Streptomyces albulus subsp.
wuyiensis, widely used as an antifungal agent in agriculture
[15]. The high-throughput capability of mass spectrometry analyses was exploited to evaluate proteomic changes occurring within
Penicillium species following treatment with plant-derived pesticides. Recently, proteomic changes occurring following treatment of
P. expansum with chitosan, used as a promising alternative for postharvest diseases management, were investigated by two-dimensional electrophoresis (2-DE) coupled to MALDI-TOF MS analysis
[16]. A comparative analysis of both mycelial and extracellular proteomes of
Penicillium janczewskii was performed to evaluate the impact on the fungus metabolism of the labdanolic acid, a terpenoid from
Cistus ladanifer [17]. Moreover, an iTRAQ-based high resolution MS approach was applied for the determination of proteomic alterations in
P. expansum spores under decanal stress
[18]. Similarly,
Penicillium digitatum proteome changes were evaluated in response to the antifungal extract produced by
Streptomyces lavendulae strain X33 with the aim of exploring the intrinsic molecular mechanism of the bacterial extract on the fungus
[19].
In this work, the molecular determinants of Penicillium digitatum response to α-sarcin and BE27, two inhibitors of protein synthesis, were investigated. We further evaluated protein changes following treatment with the commonly used fungicide thiabendazole (TBZ), a microtubule-destabilizing drug that inhibits mitosis [20] with the aim to compare P. digitatum response to biological and chemical fungicides. An advanced quantitative proteomic approach based on Tandem Mass Tag (TMT) isobaric labelling and nano-liquid chromatography coupled with high resolution tandem mass spectrometry (nanoLC MS/MS) was used to identify and quantify the expression levels of proteins from treated and untreated P. digitatum mycelia (Figure 1).
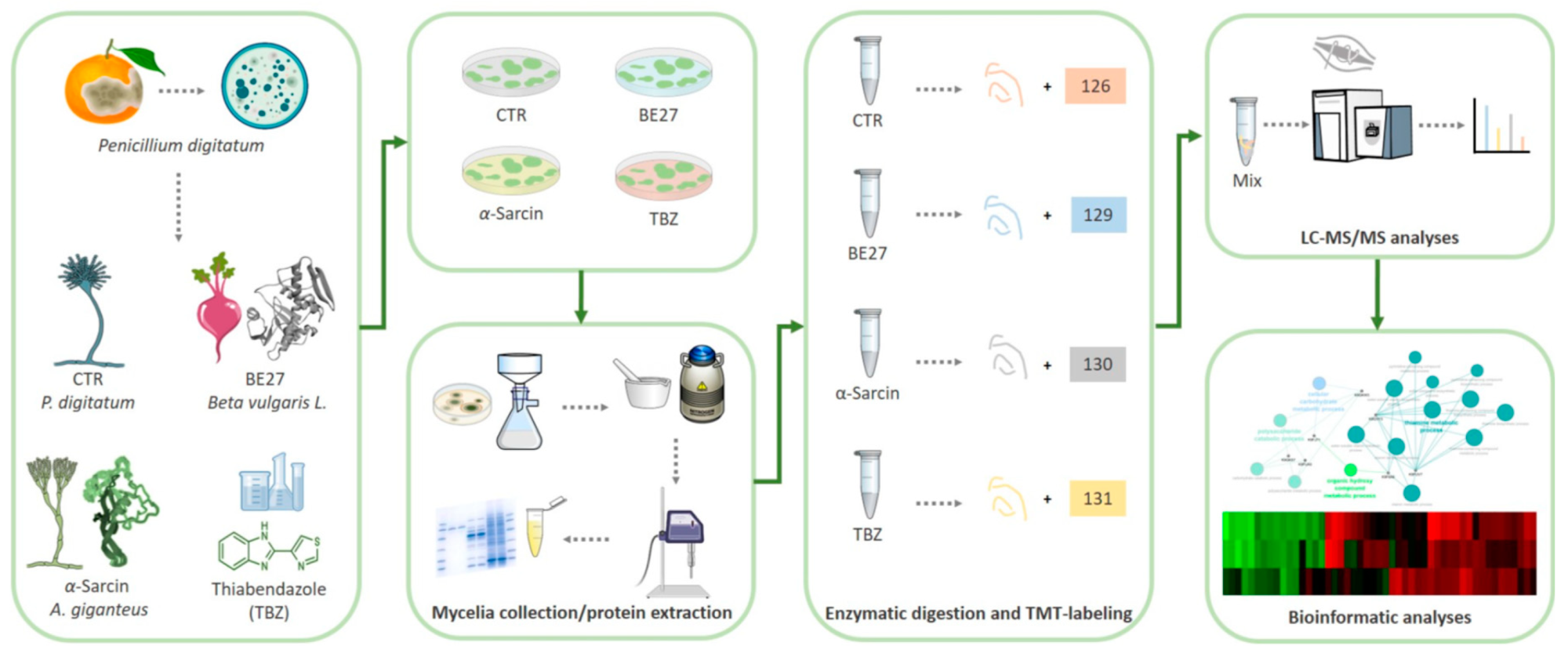
Figure 1. Schematic workflow applied for proteomic analysis of P. digitatum treated with fungicide compounds (i.e., α-sarcin, BE27 and TBZ).
Collectively, we identified candidate proteins potentially associated with α-sarcin, BE27 and TBZ treatment outcomes in P. digitatum. These were mainly involved in cell wall degradation and fungal morphogenesis, stress response, antioxidant and detoxification mechanisms and metabolic pathways. Although a similar trend in protein changes was driven by the three antifungal treatments, a distinct regulation in response to α-sarcin and BE27 treatments was also observed for a subset of proteins.
We focused primarily on differentially expressed proteins potentially impairing P. digitatum growth and virulence such as cell wall-degrading enzymes (CWDEs) and proteases. In phytopathogenic fungi, an arsenal of catalytic proteins supports nutrient acquisition, conidial formation, substrate colonization and host invasion [21]. We found several CWDEs and proteases that were differentially regulated upon protein toxins and TBZ antifungal treatments such as pectinesterases, pectate lyases, polygalacturonases, glucanases and peptidases. The importance of CWDEs in the virulence and pathogenesis of P. digitatum has been reported during the infection of oranges [22] and on postharvest citrus [23].
On the pathogen side, we observed an upregulation of CWDEs belonging to the classes of pectinesterases and pectate lyases when treated with both biofungicides and TBZ, while some differences among treatments were related to the expression levels of polygalacturonases and glucanases as well as some peptidases. These results suggest that α-sarcin and BE27 toxins may trigger a specific response in modulating cell wall integrity. In this framework, the proteins involved in cell wall morphogenesis and remodelling were also differentially regulated, such as Hydrophobin and GPI-anchored membrane protein. Hydrophobins are surface-active proteins produced by filamentous fungi. They have a role in fungal growth as structural components and fungi’s interaction with their environment. They are essential for aerial growth and the attachment of fungi to solid supports. Spores of filamentous fungi are also covered by Hydrophobins that renders the conidial surface hydrophobic and wet-resistant
[24]. In our experimental model, the upregulation of Hydrophobin may, thus, potentially also affect the observed effects on mycelium growth and morphogenesis. Other proteins that are functionally involved in maintaining cell wall integrity, such as GPI-anchored membrane proteins
[25] and C-4 methyl sterol oxidase Erg25
[26][27] were differentially regulated by both protein toxins or by BE27 alone, respectively. These sources of evidence support the idea that toxins could affect the maintenance of the
P. digitatum plasma membrane’s stability and fluidity.
Some proteins that are differentially regulated by antifungal treatments were related to energetic metabolism and amino acid metabolism, pointing to a global metabolic reprogramming during
P. digitatum’s response to antifungal treatments. A subset of these proteins is involved in the biosynthesis of vitamins and cofactors such as thiamine (Vitamin B1) and its precursor thiazole. These molecules are precursors of the active thiamine diphosphate (TPP), an essential coenzyme of several metabolic enzymes including Pyruvate decarboxylase, which was also upregulated following both protein toxins and TBZ treatments. These results implied that a response in the expression of key enzymes involved in thiamine biosynthesis is elicited, which is likely to counteract the metabolic stress triggered by the three anti-fungal agents. Interestingly, consistent with our results, thiamine metabolism was also reported to be affected by the antifungal extract of the
S. lavendulae strain X33 on the mycelial growth of
P. digitatum [19].
Treatments with fungicides also trigger a response in some
P. digitatum mediators of the redox system and stress-response homeostasis, such as the upregulation of Catalase in response to TBZ. Catalase is a well-known antioxidant enzyme produced by fungi as an infection strategy
[28]. In
P. digitatum, it was suggested that Catalase eliminates hydrogen peroxide produced by the plant as a defence mechanism
[29]. Besides the role in plant infection, in agreement with our findings, catalase was also upregulated in
P. digitatum treated with the X33 antifungal extract
[19]. Proteins related to antioxidant defence response were also differentially regulated in responses to chitosan in
P. expansum [30]. In our experimental system, further changes in the expression levels of Glyoxalase and Glutaredoxin-like proteins were elicited by α-sarcin and/or BE27, indicating that toxins incubation promoted the expression of defence-related enzymes to modulate redox homeostasis.
Another stress-response protein, downregulated following treatments with α-sarcin and BE27, was the MFS monosaccharide transporter. MFS transporters are involved in virulence by regulating the secretion of host-specific toxins or providing protection against plant defence components
[31]. Together with ABC transporters, these carriers are the most important efflux pumps involved in fungal protection against fungicides
[32][33]. More importantly, they were also reported to increase resistance to fungicides through their ability to transport a wide variety of compounds, such as toxic products
[22].
BE27 treatment alone also downregulate the expression level of the small cysteine-rich protein Antifungal protein AFP, which is endowed with a specific antifungal activity
[34][35].
P. digitatum genome sequencing allowed the identification of several potential AFP-like proteins
[36]. Although AFP constitutive expression in filamentous fungi has a negative impact on their growth and virulence, the biological role of endogenous proteins remains unclear. In other filamentous fungi such as
A. niger and
A. giganteus, it was suggested that AFPs are molecules that are important for survival under nutrient limitation
[37]. In order to trigger a strong defence response, they were also hypothesized to act as sensors, signalling or effector molecules for plasma membrane destabilization and subsequent cell lysis
[37].