Keratohyalin granules were discovered in the mid-19th century in cells that terminally differentiate to form the outer, cornified layer of the epidermis. The first indications of the composition of these structures emerged in the 1960’s from a histochemical stain for histidine, followed by radioauto-graphic evidence for a high incidence of histidine incorporation into newly synthesized proteins in cells containing the granules. Research during the next three decades revealed the structure and function of the major protein in these granules, which was initially called the ‘histidine-rich pro-tein.’ Steinert and Dale named the protein ‘filaggrin’ in 1981 because of its ability to aggregate keratin intermediate filaments. The human gene for the precursor ‘profilaggrin’ was reported in 1991 to encode 10, 11 or 12 nearly identical repeats. Remarkably, the mouse and rat genes encode up to 20 repeats. The lifetime of filaggrin is the time required for keratinocytes in the granular layer to move into the inner cornified layer. During this transition, filaggrin facilitates the collapse of corneocytes into an impermeable surface barrier. The subsequent degradation of filaggrin is as remarkable as its synthesis and the end-products aide in maintaining moisture in the cornified layer. It became apparent that ichthyosis vulgaris and atopic dermatitis were associated with the absence of this protein. McLean’s team in 2006 identified the cause of these diseases by discov-ering loss-of-function mutations in the profilaggrin gene that led to dysfunction of the epidermal surface barrier. This story illustrates the complexity in maintaining a healthy, functional surface barrier.
1. Keratohyalin Granules and Histidine
Karen Holbrook [1] commented that “morphology is often the starting point of an investigation.” Developments in microscopy during the 19th century opened morphology of the cellular world to full view. The ability to thin-section fixed tissues and innovations in selectively staining cellular components provided biologists with opportunities to study structures of tissues and the organelles within cells. Several striking observations made by histochemical and radioautographic analyses of the epidermis were found, which led to the discovery of filaggrin and its short-lived but essential functions in assembly of a healthy surface barrier.
A fascinating morphological feature forms under the cornified layer of the epidermis (Figure 1) as keratinocytes move outward from the proliferative basal layer and terminally differentiate into corneocytes that form the surface barrier of the skin. Stephen Rothman [2], recounting the early history of studies on these ‘keratohyalin granules,’ remarked that during those slower years of “wax candles and horse carriages,” there was time for “detailed and precise observations that revealed the existence of granules in the granular layer, [which were] first observed and recorded by Auffhammer (1869).” The granules were further studied by Langerhans (1873) and designated ‘keratohyalin’ in 1882 by Waldeyer. Although very active in research on the skin throughout his career, Rothman commented shortly before he died in 1963 that not much more had been learned since those early days [3]. He did not live to see the dramatic developments emerging from the investigations of these unusual structures that were already underway in several laboratories.
Seminal observations were published in the years following 1960. Reaven and Cox [4][5] traced the accumulation of zinc in the granular layer to chelation by the high density of histidine in the keratohyalin granules [6]. The granules stained an intense red color with diazotized sulfanilic acid under alkaline conditions, a coupling reaction developed by Pauly [7] for histidine (Figure 2A). Kimie Fukuyama, while a visiting scientist in I. A. Bernstein’s laboratory at the University of Michigan, began investigations of the epidermis by the incorporation of radiolabeled nucleotides into DNA and then into RNA by radioautography. She observed that, whereas DNA was synthesized only in the basal layer, incorporation of precursors into RNA occurred throughout the layers below the stratum corneum [8][9], which suggested that proteins were also synthesized in the outer layers. The surprise came when she turned to the incorporation of [3H]-amino acids. As expected, labeled phenylalanine, leucine and methionine were extensively incorporated into the dividing cells of the basal layer but minimally in the outer layers. Conversely, [3H]histidine and [3H]glycine were preferentially incorporated into the granular layer (Figure 2B) [10][11][12]. The possibility of synthesis of a unique protein exclusively in the granular layer while the cells are undergoing terminal differentiation was proposed by Bernstein, who had become interested in the epidermis as a tissue to study the process of differentiation [13]. This hypothesis called for a deeper analysis, which could only be resolved by purification of a protein enriched in histidine and glycine.
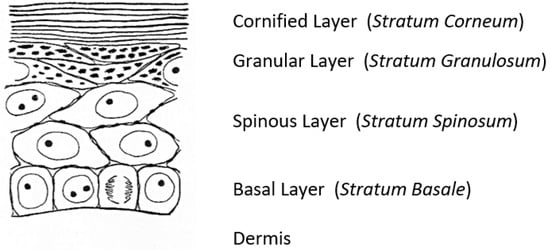
2. Discovery of the ‘Histidine-Rich’ Protein
This goal was accomplished in Bernstein’s laboratory by one of the authors (JKH) while a graduate student. A protein fraction was extracted from isolated epidermis of newborn rats that contained several-fold more histidine, and a five- to six-fold greater specific radioactivity from injected [
3H]histidine or [
3H]glycine, than the remaining bulk protein
[15][16]. Subsequent graduate students and postdoctoral fellows continued to refine the study of this protein fraction, which became known as the ‘histidine-rich protein (HRP)’ and was definitively localized to the granular layer by dissociation of the lower layers of the epidermis with tetraphenylboron
[17]. Extraction of the granular and cornified layers with 8 M urea, dialysis, lyophilization and extraction of the dried material with 0.1 N perchloric acid followed by precipitation at pH 4.5 (the protocol developed by Hoober
[14]) yielded a basic protein fraction that contained 7% histidine, 12% arginine, 16% serine and 14% glycine
[16][17][18]. The puzzling observation that 8 M urea was required for extraction of a protein inherently water soluble because of the high content of polar amino acids would become clear many years later. Ball et al.
[19] prepared a high molecular weight HRP from the granular layer that was converted to a smaller form in the stratum corneum, with kinetics of an apparent precursor/product relationship. Ugel
[20][21] extracted proteins from bovine hoof epidermis with 1 M potassium phosphate, pH 7.0, which when dialyzed formed aggregates similar to keratohyalin granules. Application of this technique to newborn rat epidermis generated keratohyalin granule-like structures that contained HRP, thereby providing definite evidence for localization of this protein in the granules
[22]. Electrophoresis of proteins in the granules revealed a main fraction with a mass of 46.5 kDa. In a study of mouse epidermis, Balmain et al.
[23] found that histidine-rich proteins with a range of 70 kDa to 120 kDa were rapidly synthesized in vitro, whereas a 27 kDa protein appeared simultaneously with the production of mature keratohyalin granules in fetal epidermis
[24]. Interestingly, the high concentration of histidine detected by the Pauly reaction did not persist as the cells moved into the cornified layer
[4][5][17]. Likewise, [
3H]histidine that was incorporated into proteins that were synthesized in the granular layer was lost as the cells moved outward
[11][19], which suggested that the protein was degraded to soluble products.
The biochemical work on keratohyalin granules that began in Bernstein’s laboratory had revealed the unusual composition of HRP, and, combined with the unconventional protocol for purification of the protein, initiated a path of research that led to characterization of a “remarkable” protein, as described by Brown and McLean
[25]. Beverly Dale introduced purification of epidermal proteins by ion-exchange chromatography in 4 M urea, with which she obtained a ‘basic protein’ with properties similar to HRP
[26]. Steinert, Dale and their colleagues
[27] succeeded in further purifying the protein to homogeneity in 8 M urea, with final steps in dilute formic acid, from newborn mouse and rat epidermis. The mouse and rat proteins were estimated by gel electrophoresis to have masses of 30 kDa and 48 kDa, respectively. However, equilibrium sedimentation in 6 M guanidine hydrochloride yielded masses of 25.8 kDa and 38.4 kDa, respectively. The purified basic proteins, with a pI greater than pH 9.5
[26][27][28], contained about 8% histidine and 12 or 14% arginine, but little, if any, lysine, valine, leucine, tyrosine, phenylalanine or tryptophan and no cysteine or methionine.
Peter Steinert and colleagues had extensively studied the intermediate filaments of keratin
[29]. When these filaments were prepared from subunits purified from mouse, bovine and hamster tissues and mixed with HRP purified from mouse stratum corneum, a rapid increase in turbidity occurred with formation of macrofibrils. Analysis of these complexes revealed 2 mol of HRP per 3 mol of keratin intermediate filament subunits. Control experiments showed that the basic protein did not interact specifically with F-actin or tubulin. These findings were the first demonstration of the function of HRP, and Steinert, Dale, and their colleagues bestowed upon the protein the name ‘filaggrin’ (fil-ăg’-grin) for its ability to aggregate keratin intermediate filaments
[27]. In contrast to filaggrin, keratin is synthesized in cells of the basal and spinous layers and becomes the bulk of the protein content in corneocytes
[30].
3. The Precursor, Profilaggrin
In 1989, Steinert’s research team at the National Institutes of Health, in collaboration with Bernstein at the University of Michigan, Croce at Temple University, Parry at Massey University and Lessin at the University of Pennsylvania, published the partial structure of the human profilaggrin gene, which contained 12 repeating units
[31]. The nucleotide and deduced amino acid sequences showed that each repeat contains 324 amino acids, with considerable sequence variation among the repeats. Each repeat of the human protein is separated by a conserved linker of seven amino acids with the sequence FLYQVST
[31], whereas the rat and mouse profilaggrins have linkers that contain the sequence VYYY
[32]. Cleavage of the protein and trimming of the linker provide filaggrin monomers with 317 amino acids and a mass of 37 kDa.
Figure 3 illustrates the structure of the human profilaggrin gene.
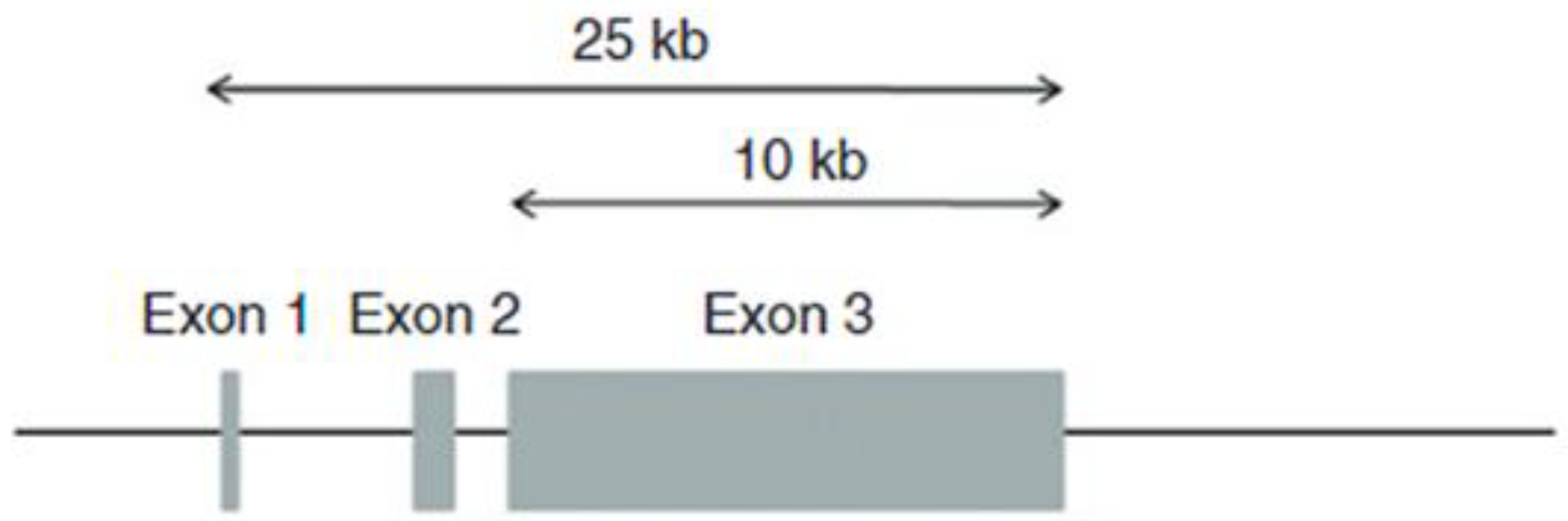
Remarkably, the mouse
[33] and rat
[34] profilaggrin genes were found to contain 20 repeats. Nearly equal amounts of two different, randomly arranged repeats, 250 and 255 amino acids in length, occur in the mouse protein, and are separated by short linker sequences. The mouse and rat proteins have 60% homology between the sequences
[35], but both have less sequence homology with the human protein.
4. The Short Life of Filaggrin
Upon synthesis, profilaggrin is heavily phosphorylated and is incorporated into keratohyalin granules. As cells of the granular layer move through the transitional zone into the inner layers of the cornified layer, the protein is dephosphorylated, and a complex group of proteases cleaves the precursor into monomeric filaggrin, which binds to keratin intermediate filaments and causes collapse of the cytoplasm into the predominant component of the flattened corneocytes
[27][36]. As the cells move outward, only the first few inner layers of the cornified layer contain filaggrin, whereas the outer layers retain keratin
[37]. The absence of histidine by histochemical analysis and [
3H] from labeled histidine in the outer cornified layers revealed that filaggrin is degraded as corneocytes proceed through the inner layers of the stratum corneum.
A flowchart that illustrates the steps in this process is provided in Figure 4 (see legend for reference numbers). The N-terminal A domain, which contains the Ca2+-binding EF-hand sequences, is cleaved from profilaggrin prior to processing of the remainder of the protein. Skin-specific retrovirus-like aspartic protease (SASPase) cleaves the linker sequences to yield active monomers that bind to keratin intermediate filaments. Knock-out of the serine proteases CAP1/Prss8 and matriptase/MT-SP1 caused incomplete processing of profilaggrin and little, if any, monomeric filaggrin, which suggested that these proteases are also involved in processing profilaggrin to the monomeric form. Upon completing its function in facilitating collapse of keratin filaments, filaggrin is further degraded by calpain 1, caspase 14 and bleomycin hydrolase to free amino acids (Figure 4B). The final mixture of amino acids, along with urocanic acid (UCA) produced by deamination of histidine, 2-pyrrolidone carboxylic acid (PCA) produced by cyclization of glutamine and other metabolic products, have been designated the ‘natural moisturizing factor (NMF)’ and aid in maintaining hydration of the stratum corneum. The activity of most of these proteases is Ca2+-dependent.
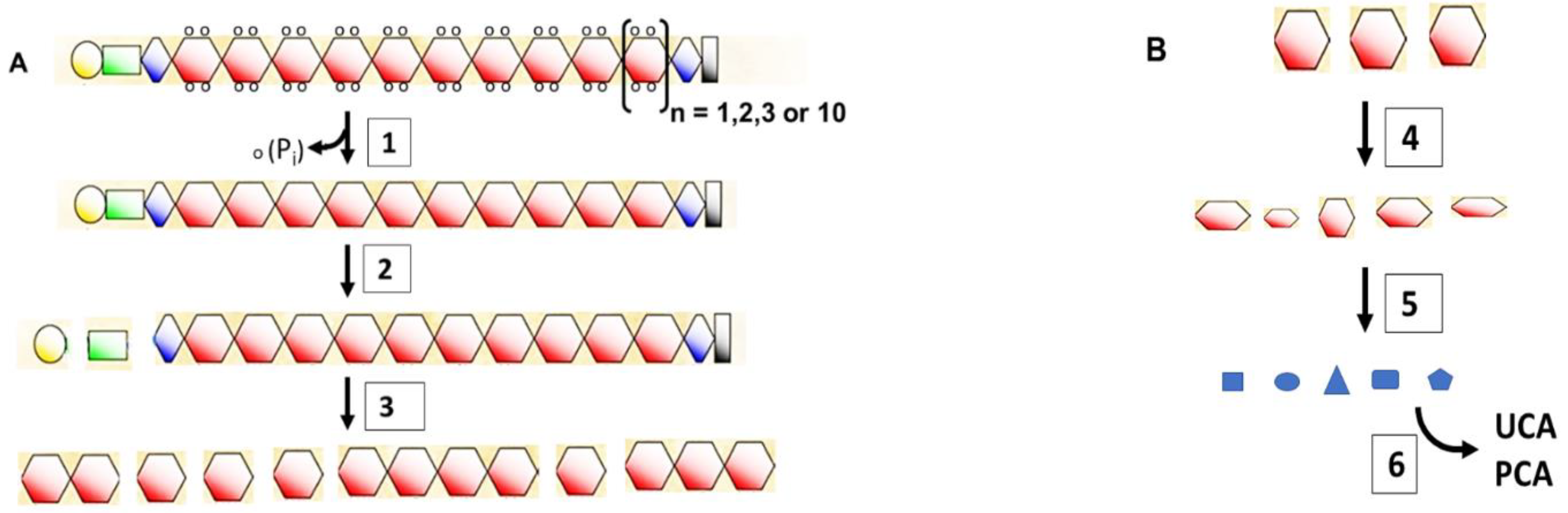
5. Mutations That Cause Loss of Filaggrin
A major discovery published in 2006 revealed the cause of the complete loss of filaggrin in ichthyosis vulgaris and atopic dermatitis (AD), diseases that result from defective formation of the surface barrier
[52]. The loosened cornified layer of the skin in these cases allows water to escape and allergens to gain access to internal cells
[53]. Irwin McLean’s team at the University of Dundee identified mutations in the first repeat in exon 3 of the profilaggrin gene (R501X generates a nonsense stop-codon, and the 2282del4 deletion also results in a stop-codon), thus revealing the genetic cause of these diseases
[25][52][53][54]. The null mutations R501X and 2282del4 are prevalent in European and Asian populations and lead to the complete absence of profilaggrin in homozygous individuals. Within the next 5 years, an extensive mutation map was generated that showed significant differences between European and Asian populations (
Figure 5). A recent analysis of 126 patients with atopic dermatologic disorders in Saudi Arabia detected 227 variants, including missense, silent, nonsense, frameshift and noncoding mutations in exon 3 of the profilaggrin gene
[55].
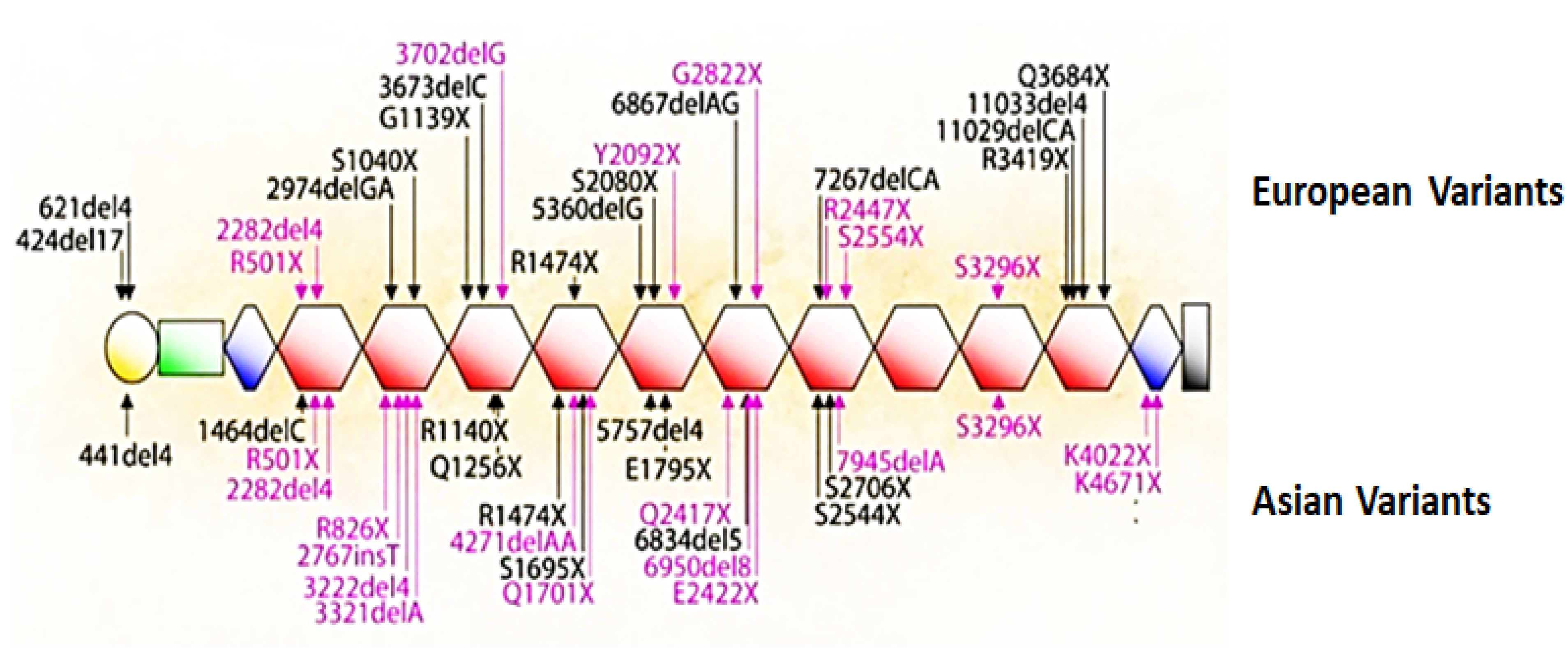
6. Keratinocyte to Corneocyte Transition
The context in which filaggrin functions is the transition of keratinocytes to corneocytes. More specifically, this transition involves formation of the cornified envelope and the conversion of cell cytoplasm to an insoluble matrix. Proteins synthesized in the granular layer are organized into a structure, referred to as the ‘cornified envelope,’ that lines the cytoplasmic surface of the cell membrane
[66][67][68][69]. Transglutaminases catalyze cross-links between these proteins, which are essential for stabilization of the complex. Three isozymes of the Ca
2+-dependent transglutaminase family, TGM1, TGM3 and TGM5, are expressed in the epidermis and function within terminally differentiating keratinocytes
[69]. An early step in formation of the cornified envelope is attachment of involucrin to the inner surface of the cell membrane by cross-links catalyzed by the essential enzyme TGM1
[67][69]. Although involucrin contains 39 repeats of a 10-amino-acid sequence, with each containing three glutamine (Q) residues, only one in this 585-amino acid protein—within the sequence ELPEQ
QVGQP (the reactive Q is underlined and bold)—is a substrate for TGM1 in vitro unless the protein is proteolytically degraded
[70]. Loricrin, an abundant protein that comprises about 80% of the cornified envelope, is synthesized in cells of the granular layer and forms oligomers with small, proline-rich proteins
[71][72]. These complexes are stabilized by TGM3 and then fixed onto the involucrin-containing scaffold by formation of cross-links catalyzed by TGM1
[66]. The keratin filament-filaggrin complex and several minor proteins, such as elafin, are added sequentially to the inner surface of the cell membrane. Keratin and a small amount of filaggrin are cross-linked to loricrin but not to each other
[72]. Cross-links, mostly to loricrin, stabilize the cornified envelope and generate the insoluble matrix of corneocytes. Mutations in the TGM1 gene cause the skin disorder lamellar ichthyosis, and
TGM1−/− knockout mice die within a few hours after birth
[67][73].
Liedén et al.
[74]and Su et al.
[75] demonstrated a remarkable increase in expression of these transglutaminases in the epidermis of patients with AD. Whereas TGM1 and TGM3 are intracellular enzymes, repair of damage to the epidermis appears to also require extracellular transglutaminase activity. TGM2 is ubiquitously expressed and, unlike other members of the family, is found in the extracellular space
[76][77][78][79]. Expression of TGM2 is induced in inflamed and wounded tissues
[76][80][81][82][83]and also by dexamethasone
[84]. TGM2 normally occurs in an inactive, closed conformation but is rapidly converted to the open, active form by injury
[80][85]. Closure of wounds is significantly impaired in TGM2 knock-out mice
[86], and processes required for wound healing and extracellular matrix remodeling are dramatically reduced when fibroblasts and macrophages are rendered TGM2-deficient by transfection with anti-sense RNA
[80][81].
Whereas the corneocytes are the “bricks” of the surface barrier, ceramides and other lipids are extruded into the extracellular space to form the “mortar”
[87][88]. Impermeability to water is provided by the extracellular lipids, as demonstrated by complete abrogation of the permeability barrier by the extraction of lipids
[89][90]. Points of contact between corneocytes occur at villus-like structures, which at their tip contain corneodesmosomes, a complex consisting of cohesion proteins corneodesmosin, desmoglein 1 and desmocollin 1
[91][92]. Corneodesmosin is synthesized in the lower granular layer of the epidermis and is secreted from keratinocytes in vesicles as a glycoprotein
[93][94]. In healthy skin, corneocytes are tightly stacked in the stratum corneum and express these villus-like structures mostly on their outermost rims (
Figure 7B). Riethmüller et al.
[95] discovered that these structures are approximately five-fold more numerous on homozygous
FLG−/− cells than on wild-type cells and cover the entire surface of the loosely packed mutant corneocytes (
Figure 7A). The less tightly stacked stratum corneum of
FLG−/− individuals is also characterized by a nearly two-fold increase in TEWL
[95]. Although important for stability of the stratum corneum, corneodesmosomes do not inhibit water flow between cells. However, TEWL could possibly be reduced if adherence of corneocytes in filaggrin-deficient patients was increased.
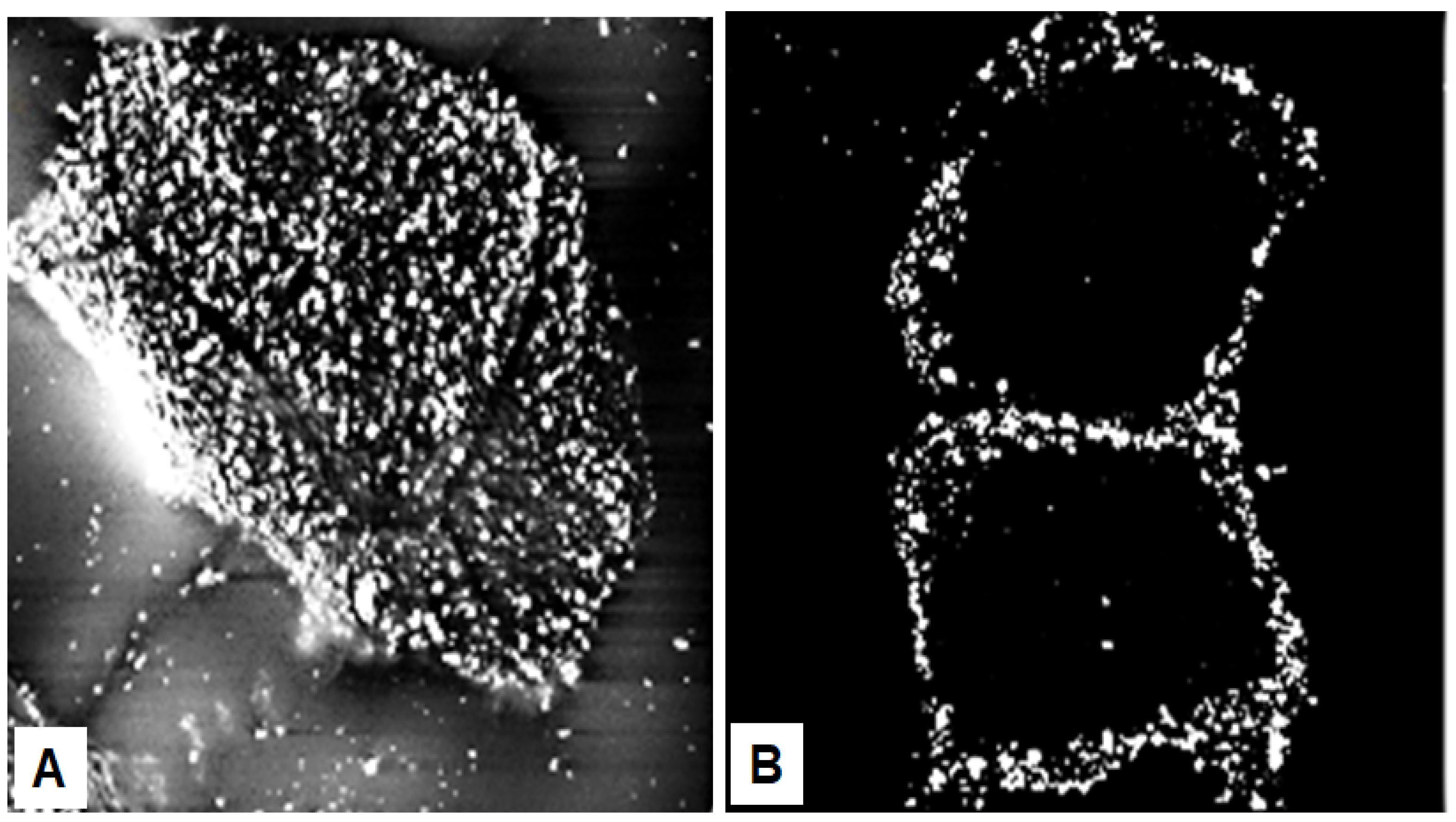