1. Introduction
Antimicrobial resistance (AMR) occurring in bacteria, viruses, fungi, and parasites has been declared by the World Health Organization (WHO) as one of the top 10 global public health and development threats facing humanity. The misuse and overuse of antimicrobials make almost all disease-causing microbes resistant to drugs commonly used to treat them
[1]. Multidrug resistance (MDR) to critical classes of antibiotics has gradually increased in nosocomial pathogens, including
Enterococcus faecium,
Staphylococcus aureus,
Klebsiella pneumoniae,
Acinetobacter baumannii,
Pseudomonas aeruginosa, and
Enterobacter spp. (which are gathered in the so-called ESKAPE group)
[2]. Currently, in Europe, AMR is estimated to be responsible for 33,000 deaths every year and the annual economic toll, covering extra healthcare costs and productivity losses, amounts to at least EUR 1.5 billion
[3][4]. Unless adequately tackled, 10 million people a year will die from drug-resistant infections by 2050, according to the predictions of the government-commissioned O’Neill report
[5].
With the decline in the discovery of new antimicrobials since 1970s, the mainstream approach for the development of new drugs to combat emerging and re-emerging resistant pathogens has focused on the modification of existing compounds. However, one key recommendation encourages stimulation of early stage drug discovery
[6]. Among emerging antimicrobial therapeutic alternatives, light-based approaches show particular promise
[7]. Phototherapy was already a common practice in ancient Greece, Egypt, and India to treat skin diseases
[8]. At the beginning of the 20th century, Oscar Raab first described the phototoxicity of the dye acridine red against
Paramecium caudatum, and Tappenier and Jesionek reported the photodynamic effects of eosin suitable for treating diverse cutaneous diseases. Since then, photodynamic therapy (PDT) is established as the administration of a non-toxic photosensitizer (PS) followed by exposure to light irradiation at an appropriate wavelength focused on an area to treat
[7].
Generally speaking, a given PS has the potential to produce reactive oxygen species (ROS) under specific conditions (
Figure 1A). Typically, it possesses a stable electronic configuration called ground state level. Following irradiation and absorption of a photon, the PS is converted from a low (fundamental) energy level (
1PS) to a “Frank Condon” short-lived, very reactive, excited singlet state
1PS*
[9][10][11]. Subsequently, the PS can lose energy by emitting fluorescence or heat via internal conversion (IC), thereby returning to its initial ground state level; alternatively, it can be converted by a so-called inter-system crossing (ISC) to a longer-living excited triplet state
3PS*. From this state, two types of chemical reaction pathways can occur, known as Type I electron transfer and Type II energy transfer, which can take place simultaneously
[12]. In the Type I reaction, the
3PS* captures an electron (e
−) from a reducing molecule (R) in its vicinity, which induces an electron transfer producing the superoxide anion radical (O
2●−) and, after the subsequent reduction, leads to the generation of more cytotoxic ROS including hydrogen peroxide (H
2O
2) and hydroxyl radical (HO
●). In the Type II reaction, a direct energy transfer occurs from the
3PS* to the ground state molecular oxygen (
3O
2) that is then converted to singlet oxygen (
1O
2). The ROS thus produced encompass O
2●−, H
2O
2, HO
●, and
1O
2, the last two being the most reactive and most cytotoxic species but also those with the shortest diffusion distance. One PS molecule can generate thousands of molecules of
1O
2, depending notably on its
1O
2 quantum yield, the surrounding environment, and the respective occurrence of Type I and Type II mechanisms
[13][10][14].
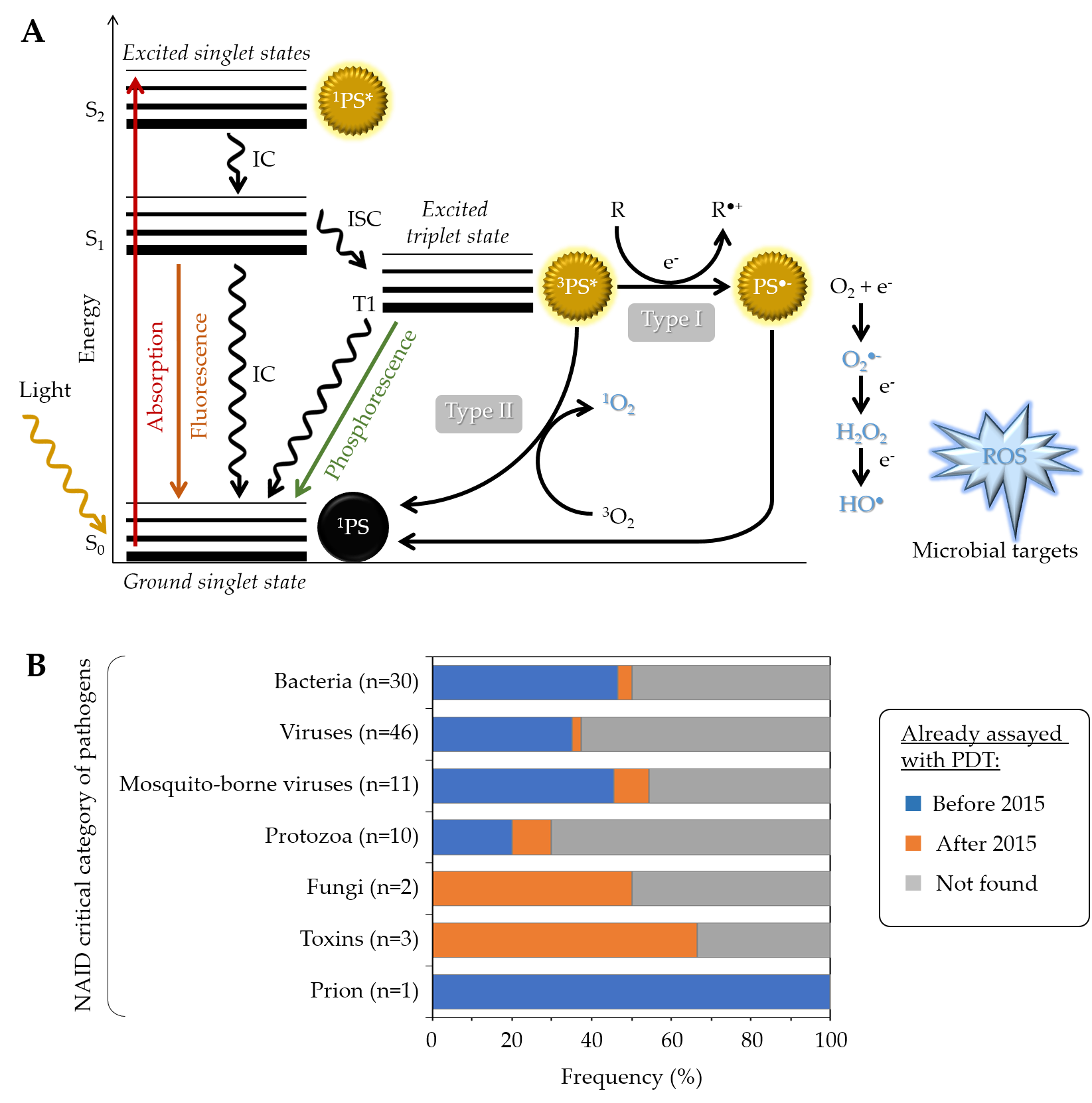
In addition to the above-mentioned Type I and Type II mechanisms, Hamblin et al. recently proposed introduction of a Type III photochemical pathway, following which the radical anion PS
•− and/or inorganic radicals formed in absence of oxygen could also lead to photoinactivation
[16]. These authors indeed identified several circumstances in which oxygen-independent photoinactivation of bacteria using specific PSs can be obtained.
While anti-cancer PDT is a clinical reality for 25 years
[17], PDT as an antimicrobial treatment was demonstrated for the first time against drug-resistant infections in the healthcare sector in the early 1990s, leading to a “photo-antimicrobial renaissance era”
[7]. Since then, the number of studies dealing with antimicrobial PDT (aPDT) has dramatically increased, emphasizing the potential of this therapeutic approach to treat a broad spectrum of microorganisms, including bacteria
[2][18][19][20][21][22][23], fungi
[24][25][26][27][28][29][30][31], viruses
[32][33][34][35][36][37][38], and parasites
[39][40][41][42][43] (
Figure 1B). Accordingly, multiple clinical trials have been conducted
[15], mostly applied to the treatment of oral infections , but also skin infections including Acne
[44][45][46], onychomycosis
[47][48], osteomyelitis
[49], or gastric ulcers
[50]. At the current stage of development, aPDT cannot address systemic infections but it holds great promise for treating localized ones and to fight AMR.
Major MDR bacteria have been found susceptible to aPDT, independently of their drug-resistance profiles and resistance is rarely reported
[51][52]. Given the multi-targeted nature of aPDT, the incidence of resistances is supposed to be very low
[53]. However, micro-organisms may be able to respond to aPDT in different ways. For instance, light response adaptation can occur in some microorganisms, such as
Escherichia coli upon exposure to blue light inducing the production of a biofilm polysaccharide colonic acid
[54]. Moreover, some PSs are substrates of efflux systems that may be overproduced; specific inhibitors of the latter can be used to restore phototoxicity
[55][56][57]. After sublethal aPDT, the biofilm-forming ability of bacteria can increase, making them less susceptible to the same treatment
[58]. Each strategy should thus be carefully examined with regard to the ability of target microorganisms to adapt and escape treatments. The latter may be noticeably reduced when using at the same time multiple antimicrobial molecular partners and modalities. Thus, aPDT evolves towards increasingly sophisticated, integrated, and innovative strategies, leading to the concept of combinatory aPDT (c-aPDT)
[15].
Following excellent earlier authoritative reviews
[13][59][60][61], most recent developments since 2015 in the field of aPDT are highlighted herein, with a special emphasis on combinatory approaches
[15]. It should be noticed that aPDT is sometimes referred to as photodynamic antimicrobial chemotherapy, light-based antimicrobial therapy, photo-controlled antimicrobial therapy, or antimicrobial photo-inactivation. All these synonyms were considered herein to present (i) a state of the art with recent developments in photo(nano)systems, (ii) the implementation of multicomponent nanotechnologies and recent molecular engineering, and (iii) the exploration of combinatory aPDT approaches towards possible future therapeutic innovations.
This entry is adapted from 10.3390/pharmaceutics13121995.
5. Discussion
Antimicrobial PDT has the potential to fight against a wide spectrum of infective agents, including those resistant to conventional antimicrobials, under non-clinical and clinical settings. Rather than replacement, aPDT may be a complementary approach to reduce the use of current, especially last resort, antimicrobials. This review aims to give a non-exhaustive overview of the diversity and richness of synthetic, natural, or hybrid single PSs and aPDT nanosystems that were recently reported, with respect to their specific advantages, limitations, and possible evolutions. It is noteworthy that many systems and strategies primarily developed for anticancer PDT have been or could be applied—per se or following adaptations—to antimicrobial applications.
Beyond the “chemical space” that can be explored with individual PSs, versatile combinations with other compounds can allow the design of multimodular/multimodal systems. Along this line, the various PSs available may be considered as “basic ingredients”. Apart from offering alternative possibilities for overcoming the most common limitations of PSs (i.e., solubility, delivery, and specificity), reasons for implementing PSs in complex systems can be related to (i) the ability to target several types of pathogens at once (extended antimicrobial spectrum), (ii) synergistic antimicrobial effects, (iii) reduction in the dose of each combined component, (iv) beneficial effects in severe poly-pathogenic infections, and (v) reduction in the risk of resistance emergence.
In addition to the many possible variations concerning PSs, optimizations can also consider other critical parameters in PDT, namely light irradiation and oxygenation. The latter was considered for a long time as an indispensable component. However, control of the oxygen level in the aPDT system is questionable. Indeed, additional oxygen-independent phototoxic mechanisms have been reported, for example with psoralens, which can produce more effective aPDT without oxygen
[16]. Furthermore, recent studies suggest that various strategies could be used to reduce or bypass the limitations of oxygen and light supplies (read below). All combined, optimizations targeting not only the PSs, but also light irradiation and oxygen supply, could allow to evolve toward integrative, highly sophisticated, antimicrobial photodynamic therapy.
Light irradiation and its various modalities have been reviewed in depth by different authors
[13][454][455][456]. Typically, the irradiation in PDT occurs in the UV (200–400 nm) or in the visible light (400–700 nm) with a power of ≤ 100 mW
[455]. However, the low light-penetration depth (around mm) and possible occurrence of tissue photodamage limit the applicability of this spectral range for PDT. This can be circumvented by application of a near-infrared (NIR) irradiation (750–1100 nm), particularly via a two-photon excitation, which is emerging for PDT applications
[208][457]. Being a third-order nonlinear optic phenomenon and corresponding to the simultaneous absorption of two photons with half the resonant energy, it allows deeper penetration in biological tissues (around 2 cm), lower scattering losses, and a three-dimensional spatial resolution
[458]. Light sources are also constantly improving. Laser is an exceptional source of radiation, capable of producing extremely fine spectral bands, intense, coherent electromagnetic fields ranging from NIR to UV. In comparison, LEDs feature other advantages, notably the possibility to be arranged in many ways, in large quantity, for irradiating wide areas while inducing negligible heating
[454][455].
Considering that light-emitted sources can become a brake to any PDT applications, several promising options have been envisaged quite recently. Notably, Blum et al. have identified a series of “self-exiting way” that allows to abolish the need of light to achieve efficient PDT
[459]. Among them, chemiluminescence was extensively investigated by using luminol or luciferase energy transfer to induce a chemiexcitation of PS as antibacterial therapeutics
[460]. In addition, other methods may be based on Cherenkov radiation, which occurs when an emitted charged particle, such as an electron, moves with high speed through a dielectric medium, such as water. Thus, the polarization of electrons in the medium produces electromagnetic waves in the visible wavelengths that could activate PDT reaction. Another way to induce Cherenkov radiation is to use radioactive isotopes with high beta emissions (e.g.,
18F,
64Cu, or
68Ga) as an electronic excitation source
[459]. The relevance of such approaches for the design of a self-induced aPDT system remains to be evaluated. In addition, another external source of excitation, such as microwaves, could activate photoactive molecules such as Fe
3O
4 when they are complexed with carbon nanotubes. This was recently evaluated by Qiao et al. for treating MRSA-infected osteomyelitis
[23]. Furthermore, X-ray as an activated source can also facilitate the activation of the PDT system by transferring energy harvested from X-ray irradiation to the PS used
[461]. Kamanli et al. compared pulse and superpulse radiation modes, showing that the latter is more effective to produce
1O
2 and
S. aureus eradication than the former
[462].
Oxygen is also a critical limiting factor that determines PDT efficiency, especially in poorly oxygenated environments. However, it is noteworthy that PDT can be achieved in cancers that typically feature a low oxygenation rate. To alleviate the possible limitations of oxygen supply in PDT systems, several strategies have also been recently considered. One is based on catalase grafting to achieve oxygen self-sufficient NPs in order to convert H
2O
2 into available dissolved oxygen in the tumor environment. The abundant ROS in tumors compared with normal tissues provide a coherent substrate for catalase and thus allows an improvement of PDT activity
[463]. Some multifunctional nanomaterials, called nanozymes, can also be used in combination with PSs to achieve a catalase-like activity supplying an oxygen source for the PS functioning
[464][465]. In addition, it was also reported that noble metal NPs – such as Ru, Pt, and Au NPs – exhibit catalase-like nanozyme activities
[466]. The use of catalases or nanozymes may have the crucial role of oxygen helpers in aPDT for treating deep infections.
The possibilities to design combinatorial aPDT strategies seem unlimited. Those presented in this review partly overlap with the description of aPDT systems, showing the complexity of any classification process. Awareness and caution may be raised about some sort of “paradox” or “dilemma”; indeed, while the seemingly boundless collection of chemical options and modular tools to develop nanoscale aPDT therapeutics implicitly defines extensive design flexibility, it staggeringly complicates and bewilders at the same time the optimization process aiming to compare, rationalize, and identify the “best” option for each application. Along this line, data concerning structure-activity relationships are usually missing, with not many studies yet dedicated to this matter
[52][65][75][467]. Similar to the CLSI guidelines defined for antibiotics, uniform research methodologies would be useful to assay aPDT systems under well-defined standards and guidelines, considering notably (i) illumination settings, (ii) positive controls
[468], (iii) microorganisms and cell lines relevant for a given application, and (iv) assessment of antimicrobial efficacy; this would guarantee better-conducted preclinical and clinical trials of aPDT systems used as mono or combinatory therapy. Furthermore, a public database compiling the efficacy/side effects of various systems would also be useful, facilitating meta-analyses for delineating (quantitative) structure/activity relationships and computational simulations
[469].
Finally, in view of translation to clinical practice, a series of precautions and potential limitations must also be considered, especially when dealing with combinatory strategies. Beside possible reciprocal interferences (antagonisms) between combined partners, safety and specificity parameters must also be carefully examined. One main advantage of aPDT is the possibility to control the production of ROS thanks to the use of nontoxic PSs triggered with inducers (specific light and free oxygen) and/or enhancers. The vast majority of PSs are considered safe and dark cytotoxic side effects toward non-target eukaryotic cells are rarely reported. However, in many cases, more studies are needed for examining—beyond potential side-effects—other parameters such as bioavailability, biodispersion, persistence in host cells and body, and elimination pathways. With regard to combinatory strategies, it is noteworthy that studies conducted to date were mostly based on in vitro evaluation or using animal models bearing well-defined sites of infection. Thus, much more data in preclinical and clinical settings are required to support the actual potential of such strategies. Moreover, considering that many microbial infections are systemic, the use of modalities such as sonodynamic therapy or electrochemotherapy is at present not realistically feasible. Therefore, in spite of significant progress and real promise, further important work/innovations are needed to effectively broaden the range of infectious conditions that could be treated via aPDT approaches. Lastly, cost effectiveness of multiplexed aPDT therapies over monomodal conventional antimicrobial agents is another crucial point to be considered in view of clinical applications. Potent, but too expensive solutions may fail to be used in practice, especially in under-developed countries where conventional antimicrobials will continue to be used, allowing microorganisms to increase in resistance.