Medulloblastoma is the most prevalent malignant brain tumor in children, while it accounts for only 1–2% of adult brain tumors. Recognized as a biologically heterogeneous disease, the World Health Organization (WHO) considers there to be four molecular subgroups: wingless-activated (WNT), sonic hedgehog-activated (SHH); Group 3; and Group 4. Recently, the picture became more complex when 12 different medulloblastoma subtypes were described, including two WNT subtypes, four SHH subtypes, three group 3 subtypes, and three group 4 subtypes, with each subgroup being characterized by specific mutations, copy number variations, transcriptomic/methylomic profiles, and clinical outcomes. For the SHH subgroup MB, germline or somatic mutations and a copy-number variation are the common drivers that affect critical genes involved in SHH signaling, including PTCH1 (patched 1 homologue), SUFU (suppressor of fused homologue), and SMO (smoothened), among others
1. Introduction
The most common genetic events, which occur in both pediatric and adult tumors, are loss-of-function, mutations, or deletions in PTCH1 and SUFU , which act as negative regulators of SHH signaling [1][2][3]. Activation of mutations and amplification of SMO or GLI2 (glioma associated oncogene homologue 2) also lead to constitutive activation of the SHH pathway [4][5]. Germline and somatic TP53 mutations predominantly coincident with GLI2 amplifications and are found exclusively in children between the ages of 8 and 17 years [6][7][8][9]. Somatic TERT (telomerase) promoter hotspot mutations are also associated with the SHH subgroup [10][11]. Mutation of PTEN (phosphatase and tensin homolog) is found in more than 5% of human SHH subgroup MB cases and is associated with decreased expression of PTEN mRNA and proteins in the cerebellum [9][12]. In addition, genetically engineered mouse models (GEMMs) carrying mutations/overexpression of those genes have also been developed to study this medulloblastoma subgroup [13][14][15].
Medulloblastoma can also be viewed through the lens of the tumor microenvironment (TME), and its multiple roles in cancer offer an interesting way to identify the critical steps regulating medulloblastoma biology, disease progression, and overall survival [16][17][18][19][20][21][22][23][24][25][26][27][28][29]. In addition to tumor cells, the tumor microenvironment is characterized by diverse cell populations, including stem-like cells and tumor-associated components such as blood vessels [18], immune cells [24][25], neurons, endothelial cells, microglia [26], macrophages [27][28], and astrocytes [17][19][20][21][29]. The communication between these unique collections of cell types is implicated in therapy resistance [30][31][32], immune infiltration, and inflammation [28]. Since tumor-associated cells could be the focus of therapeutic vulnerability, a comprehensive understanding of the interactions between the tumor cells and the tumor-associated components may provide new opportunities for targeted discoveries. In the SHH subgroup MB, recent studies have highlighted that the cellular diversity within tumors has a critical role in supporting the growth of tumor cells and the robustness of cancer [17][19][21][25][27][28][29][33][34]. In MB-prone mice with a SMO mutation, the TME contains tumor cell types that exist across a spectrum of differentiation states and tumor-derived cells that express makers for astrocytic and oligodendrocytic precursors [35]. This suggests that even in a tumor with a single pathway-activation mutation, diverse mechanisms may drive tumor growth, demonstrating the need to target multiple pathways simultaneously for therapeutic effectiveness.
Astrocytes and the Medulloblastoma Microenvironment: The New Player within the Complex Ecosystem
Due to increasing evidence of an association between wound healing and the development of tumors, recent studies have investigated the complex functions of astrocytes involved in the support of medulloblastoma growth, as these specialized glial cells are involved in the functional recovery of the central nervous system (CNS)
[17][20][21][36][37][38][39][40][41][42][43][44][45][46][47][48][49][50][51][52]. Astrocytes are specialized and heterogeneous cells that are essential modulators of local blood flow as well as being involved in the maintenance of homeostasis of extracellular fluids, ions, and transmitters
[49][50]. In a healthy CNS, these glial cells participate in synaptic function and plasticity among other dynamic activities that are crucial for the neural circuit and neurological function and behavior
[49][50]. In this context, recent studies have identified SHH signaling as an essential regulator of the molecular identity and functional properties of astrocytes
[51][52]. Under normal conditions, astrocytes express the components of the SHH pathway, but do not secrete the SHH protein
[53][54]. Recent in vivo studies have shown that the SHH pathway is active in astrocytes of the mature forebrain through the SHH transduction system, which includes the receptor PTCH1 as well as GLI transcription factors
[54][55]. Others studies have also demonstrated that the SHH protein is mainly produced by neurons in several brain areas, including dopaminergic neurons
[56], the Purkinje cells and mossy cells in the hippocampus, but not in astrocytes or oligodendrocytes
[57]. In addition, under physiological stress or pathological conditions, it has been reported that astrocytes may be able to produce and become powerful sources of the SHH protein
[58][59][60][61].
In the cerebellum, specialized, unipolar astrocytes called Bergmann glia (BG) have been shown to be capable of responding to the Purkinje-derived SHH protein from the postnatal stage through to adulthood
[62]. Mice in which
SMO is postnatally ablated in BG demonstrate reduced proliferation of granule cell precursors (GCP) and increased differentiation accompanied by a loss of SHH activity. In these animals, WNT signaling is ectopically elevated in GCP, suggesting that this pathway is involved in cross-talk with the SHH pathway, which helps to regulate GCP proliferation
[62].
Astrocyte reactivity (AR)
[38][39][40][41][42][43][44][45][49][51], an ubiquitous, complex, and multistage process, is known to be involved in different CNS pathologies, including trauma
[39], inflammation
[36], stem-cell repair
[41], regeneration
[42], peripheral metabolic disorders
[43], neurodegenerative diseases
[40][44], and tumor progression
[34][45][47][63][64][65]. In the context of brain metastasis, reactive astrocytes have a dual role: they limit disease progression during the early stages and, later on, foster tumor growth
[45]. During tumor progression, reactive astrocytes are key components of the microenvironment, and their function and crosstalk with other components of the TME have been targets of neuro-oncology research
[17][21][33][63][64][65]. Astrocytes can act through paracrine secretion of degradative enzymes, cytokines, chemokines, and growth factors
[36] and have multiple primary and branching endfeet that interact with tumor cells, facilitating growth, proliferation, survival, and invasion. Recent studies have demonstrated that, in brain tumors, astrocytes secrete cytokines and trophic factors and contribute to tumor growth, metastasis, and resistance to current therapy
[41][43]. In primary gliomas and brain metastases, astrocytes establish gap junctions with tumor cells, and these functional connections are regulated by signaling molecules, such as connexin
[43]. In response to these non-cell-autonomous stimuli, astrocytes can produce a multitude of molecular signals that can, in turn, influence many different neural and non-neural cell types, including cells involved in innate immune responses
[63]. In parasite infections, astrocytes secrete the SHH protein which, in turn, induces the production of GRP78, an endoplasmic reticulum (ER) chaperone from the heat shock protein family
[20]. Under ER stress, it is believed that the activation of
GRP78 may increase cell survival through the unfolded protein response and may also protect cells from ER-stress-induced apoptosis by activating
Bcl-2 and inhibiting
Bak,
Bax,
Caspase, and
CHOP [20]. In fact, astrocytes facilitate the formation of medulloblastoma tumoroids by secreting SHH proteins and generating the astrocyte-derived extracellular matrix
[29].
The roles of astrocytes in the medulloblastoma microenvironment have been investigated, and studies have demonstrated that astrocytes secrete CD133, a key cancer stem cell marker that is involved in medulloblastoma tumorigenicity and alters gene expression, increasing invasion and adhesion by medulloblastoma cells
[48]. Astrocytes can also have a direct influence on brain tumor stem cells that are activated by several ligands, including SHH, which enriches the stem cell population
[66]. These interactions are bi-directional, and tumor stem cells can provide signals that affect the surrounding astrocytes
[65].
2. Novel Targets and Therapeutic Opportunities for Medulloblastoma: A Potential Application of Astrocytes-SHH Medulloblastoma Cross-Talk Research
Current understating of the contribution of the TME to the growth of tumor cells has shifted the focus of neuro-oncology research, moving from exclusively targeting tumoral cells to targeting the tumor microenvironment or signals coming from it, as well as the interactions between them
[67][68][21][29][33][66][69]. From multiple studies, it has become clear that the interplay between tumor cells and cells of the tumor microenvironment orchestrate events that are critical to tumor progression, and in this way, many cellular and molecular elements of the microenvironment are emerging as attractive targets for therapeutic strategies
[17][23][24][29][31][70]. Although GCPs are the most studied cells regarding the origin of SHH MB and have been the focus of the search for targets in the medulloblastoma for some time, protein receptors and peptide factors from other cellular sources that impact SHH subgroup MB have attracted the attention of researchers more recently.
The G protein-coupled receptor (GPCR) family of proteins is widely dysregulated in cancer and yet is underexploited in oncology. Recent studies have shown that GPCRs can play multiple roles in cancer progression, including proliferation, survival, angiogenesis, metastasis, therapy resistance, and immune evasion upon activation by ligands produced by cancer cells or through the multiplicity of cells within the tumor stroma
[69]. The mitogenic ciliary functions of G-protein coupled receptor 37-like 1 (GPR37L1) in SHH–SMO signaling are particularly attractive to target cancer via the tumor microenvironment. The GPR37L1, an orphan G-protein-coupled receptor is a selective marker of cerebellar BG astrocytes
[70][71], and it specifically colocalizes and interacts with the PTCH1 protein in discrete areas of these Bergmann glia cell membranes in newborn mice
[70]. The Bergmann glial cells possess the primary cilia (PC), which are antenna-like organelles required for sensing and transducing extracellular stimuli
[72]. These PC are essential for the regulation of several signaling pathways, such as SHH and WNT, and they can promote tumorigenesis in medulloblastoma
[72][73]. Primary cilia have been reliably detected in all cells of pre-neoplastic MB in
PTCH1+/− mice
[19]. Thus, the specific detection of primary cilia could be usefully applied for the study of early, pre-neoplastic MB lesion s
[73].
GPR37l1−/− mice present with precocious Bergmann glia, Purkinje neuron maturation, and increased levels of Purkinje secreted SHH protein, as well as SMO and the intracellular effectors of the SHH-SMO cascade, MYCN and GLI2
[70]. In cerebellar primary astrocyte cultures from
GPR37l1−/− mouse pups, these astrocytes displayed striking increases in proliferative activity, PTCH1 protein expression and internalization, intracellular cholesterol content, and ciliary localization of SMO, as well as marked production of active SHH signaling
[74]. Similar effects were reproduced by treating wild-type astrocytes with a putative prosaptide ligand of the GPR37l1 receptor
[74]. Using
GPR37l1−/−Ptch1+/− mice, Di Pietro et al. (2019) showed that genetic ablation of
GPR37L1 in this medulloblastoma-prone mouse model can reduce the occurrence and severity of postnatal tumors
[19] (
Figure 1). These authors speculated that this receptor could be involved in the process of BG modulation of SHH production by Purkinje neurons and suggested the involvement of WNT3, a specific inhibitor of SHH-induced neuronal mitogenesis
[19]. As GPCRs are the most “druggable” class of proteins currently known, the GPR37L1 receptors have become a highly valuable target for the development of novel therapies, and their use as a specific blocking agent may be an important target for medulloblastoma treatment.
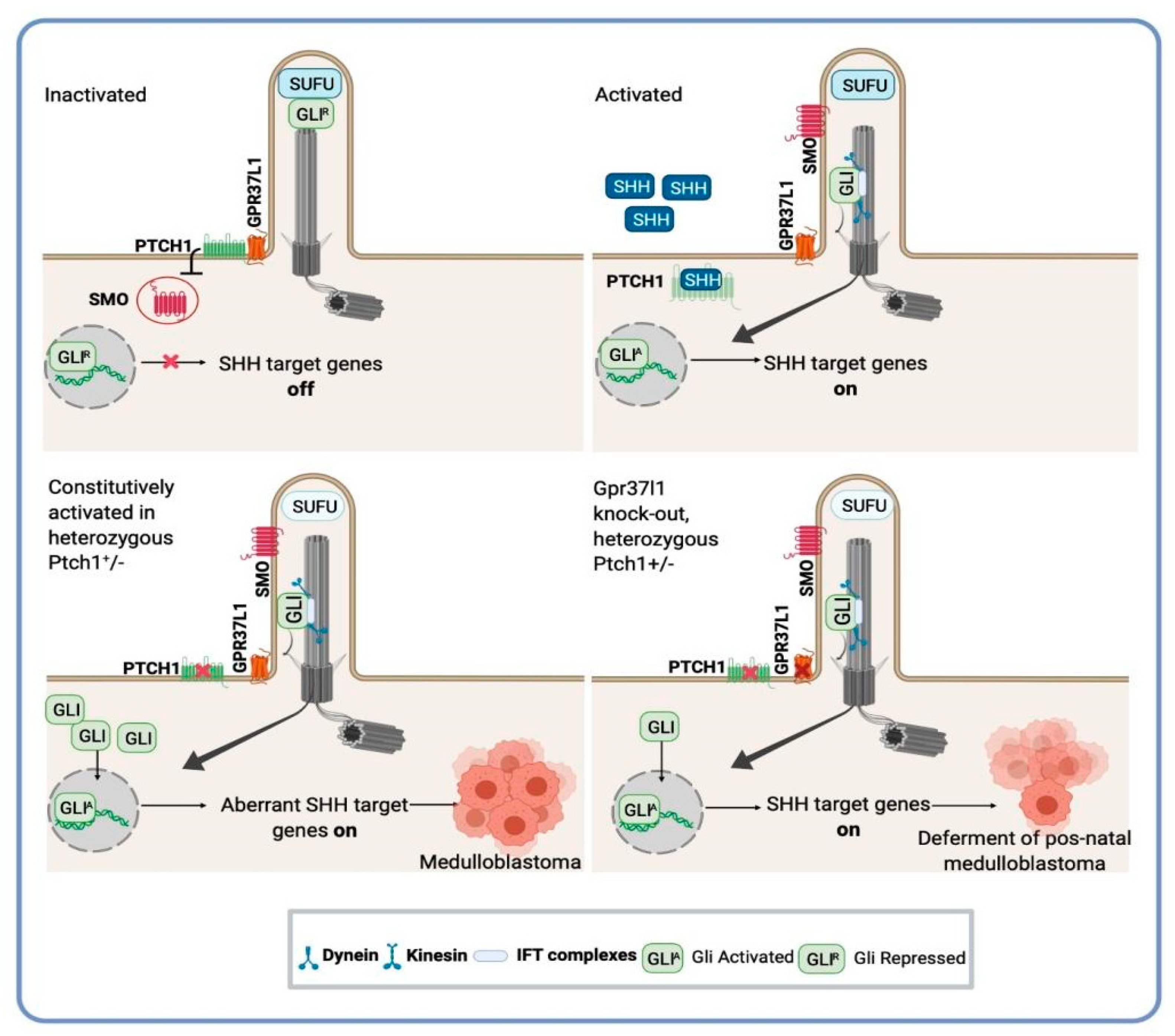
Figure 1. In the absence of the sonic hedgehog ligand (SHH), the negative regulator PTCH1 is present on the ciliary membrane. In this state (Inactivated), suppressor of fused (SUFU) forms a complex with the GLI transcription factors in the periciliary region. SHH binding to PTCH1 (Activated) induces its translocation away from the cilium and promotes the entry of the activating receptor SMO. This process allows the migration of active GLI (GLI
A) into the nucleus where the transcription of SHH target genes is activated. Mice heterozygous for loss-of-function
PTCH1 mutations have a higher incidence of medulloblastoma
[13][75][76]. In a
GPR37l1−/-Ptch1+/− mouse model, the lack of
GPR37L1 reduced the postnatal tumor occurrence of tumors and decreased the incidence of more aggressive tumor types
[19].
Regarding the critical tumor–stroma interaction related to SHH subgroup MB, Snuderl et al. showed that stromal cells produce Placental growth factor (PlGF), a member of the vascular endothelial growth factor (VEGF) family, which is stimulated via paracrine SHH ligand secretion by the tumor cells
[77]. In vitro results have demonstrated that PlGF, its receptor neuropilin 1 (Nrp1), and the MAPK signaling axis are critical for the survival of medulloblastoma cells, and in
Smo/Smo transgenic mice, the blockade of PlGF with anti-PlGF antibodies was associated with significantly smaller tumors
[77]. Together, these findings provide insight into the roles of SHH, PlGF, and Nrp1 in SHH subgroup MB, and as PlGF is dispensable during development, they support the idea that this SHH tumor and PlGF interaction may be used as a therapeutic approach for this pediatric tumor.
Added to the findings highlighted here, the effects of SHH protein secretion by tumor astrocytes on tumor progression and the adaptive transdifferentiation of the tumor and its reliance on astrocytic signals open new perspectives for discovering multiple potential therapeutic targets within the tumor-associated glial cells. With the large amount of genetic and epigenetic data from the subsets of human medulloblastoma, the design of future medulloblastoma therapies must be based on the identification of genes that are specifically expressed in these tumor-associated astrocytes.