Glioblastoma (GBM) is the primary cancer with the highest incidence within the central nervous system. Natural killer (NK) cells are innate lymphocytes that play an important role in immunosurveillance, acting alongside other immune cells in the response against various types of malignant tumors and the prevention of metastasis. NK cells are being exploited in many ways to treat cancer. The broad arsenal of NK-based therapies includes adoptive transfer of in vitro expanded and activated cells, genetically engineered cells to contain chimeric antigen receptors (CAR-NKs), in vivo stimulation of NK cells (by cytokine therapy, checkpoint blockade therapies, etc.), and tumor-specific antibody-guided NK cells, among others.
1. Introduction
Glioblastoma (GBM) considerably reduces patients’ quality of life [1]. Its current treatment—including maximal surgical resection, adjuvant radiotherapy, and chemotherapy with temozolomide—significantly improves patient survival [2]. However, this therapeutic regimen is still not enough, as half of the patients die within approximately 15 months after diagnosis [3], and less than 5% of them remain alive after 5 years [4]. Relapse occurs frequently, and death is inevitable.
A broad arsenal of treatments has been established to control or potentially cure neoplasms; more recently, immunotherapy has emerged as a new therapeutic pillar in oncology. Within this treatment modality, most strategies have been centered on either antibodies or T cells, but lately, new studies have sought to take advantage of the antitumor activity of natural killer (NK) cells.
2. Natural Killer Cells
Discovered in the 1970s during cytotoxicity studies of lymphocytes, NK cells were initially characterized as large granular lymphocytes with “natural” cytotoxicity, since they did not require prior exposure to their targets in order to perform their cytotoxic activity
[5][6][7]. They were also defined as “null” cells, considering that they were non-adherent cells in which the genes encoding antigen receptors were not reorganized, resulting in the Ig
− phenotype and in their inability to perform rosette formation with erythrocytes (non-B or -T cells)
[8]. Finally, NK cells were understood as cells with cytotoxic capacity against a wide range of target cells, but also as cells that play significant roles in inflammation and in innate and acquired immune responses, via their production of chemokines and cytokines
[9][10][11].
Kiessling and Kärre’s studies paved the way for the formulation of the “missing self” hypothesis for the target recognition of NK cells. These works demonstrated that murine lymphoma cells with low or absent expression of genes encoded by the major histocompatibility complex (MHC) class I were lysed very efficiently by NK cells, while cells expressing such molecules were resistant to lysis
[12][13]. A conclusion of these experiments and a necessary factor for the “missing self” model is that NK cells should express at least one specific receptor for MHC-encoded class I molecules (MHC I) in order to be able to detect the “self” and its absence.
Despite NK cells being classified as innate cells, NK cell responses can exhibit the adaptive phenotype of immunological memory or trained immunity under circumstances such as viral infections, hapten challenge, and stimulation with IL-12, IL-15, and IL-18 cytokines. Interestingly, memory or “adaptive” NK cells present a long lifespan and perform improved responses upon re-stimulation
[14].
NK cell development occurs in the bone marrow, and is thought to be a linear process (controversies remain at this point
[15]) with multiple stages, characterized by different membrane markers (
Figure 1); it starts from a hematopoietic stem cell (HSC) that self-renews and differentiates into a compromised multipotent lymphoid progenitor (MLP). This gives rise to the common lymphoid progenitor (CLP)—a cell with the potential to differentiate between the lineages of B, T, and NK lymphocytes
[16][17][18][19][20][21].
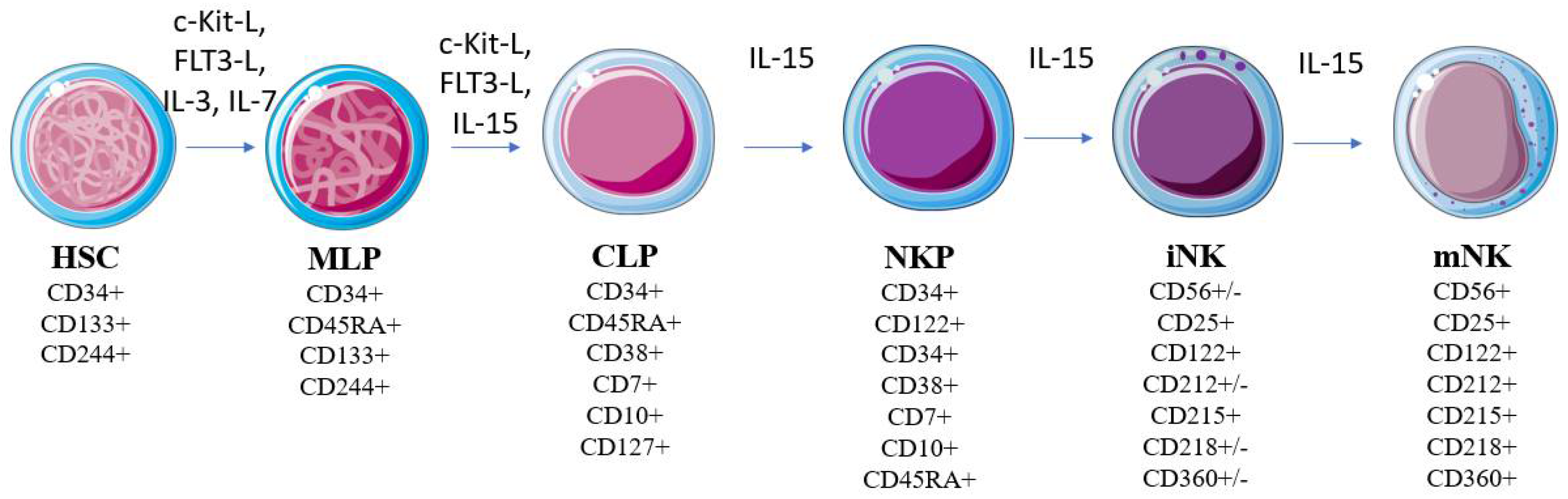
Activating receptors include natural cytotoxicity receptors (NCRs), such as NKp30, NKp44, and NKp46. Although most of the endogenous ligands of these receptors are unknown, it has been noted in recent years that NCR interactions are extremely heterogeneous, and may involve viral ligands, proteins expressed upon stress, surface glycoproteins, altered matrix molecules, surface-exposed nuclear molecules, and enzymatically released ligands
[22][23]. Other activating receptors include the FcγRIII receptor (CD16), which recognizes IgG antibodies
[24], and toll-like receptors, which recognize molecular patterns associated with damage and pathogens (DAMPs and PAMPs, respectively)
[25].
3. NK Cells in Immunosurveillance against Cancer
At least in murine models, NK cells play a central role in immunosurveillance, acting against established tumors and in the prevention of metastasis, conferring them an attractive role in immune strategies aiming to control the progression of tumors and potentially eradicating them
[26][27]. Their participation in tumor immunosurveillance has been evidenced in several studies, and is well established in murine models, where blocking the activity of effector mechanisms shared by NK cells—such as perforin, TRAIL, and IFN-γ—leads to a higher incidence and/or development of tumors when compared to wild animals
[28][29][30][31][32][33]. Likewise, and pointing more specifically to NK cells, their depletion by treatment with monoclonal antibodies to asialo-GM1 or NK1.1 increases the susceptibility of mice to chemically induced fibrosarcomas
[34].
Despite providing strong evidence for a significant role of NK cells in immunosurveillance, these are studies in murine models, and their equivalence in humans could be questioned. In this sense, a series of observations can be brought to argue in favor of an equivalent role of NK cells in humans. Initially, it must be recognized that NK cells from healthy individuals do exert ex vivo antitumor activity against cells of numerous primary tumor lineages, demonstrating the existence of spontaneous cytotoxicity against human tumors
[35].
The roles of the immune system’s different components in immunosurveillance can be inferred by observing patients with specific immunodeficiencies. However, for the establishment of the role of NK cells, the rarity of well-defined and specific immunodeficiencies of NK cells is a major obstacle
[36].
3.1. Tumor Cell Recognition
NK cells can kill cancer cells while maintaining tolerance against healthy cells
[13]. As already mentioned, any selective recognition by NK cells depends on the signals integrated by their activating and inhibitory receptors. Unlike most healthy cells, tumor cells may have low or reduced expression levels of the MHC-I molecules on their surface; this prevents the engagement of inhibitory receptors—such as KIR and NKG2A receptors—which would enable NK cell activation over the target cell
[37][38][39].
3.2. Antitumor Functions
Upon activation, NK cells can exert antitumor activities in two ways: by directly killing malignant cells, or by modulating the activity of other leukocytes (
Figure 2). The direct killing is thought to be mediated mainly by perforin/granzyme-dependent cytotoxicity, as noted in numerous experimental models
[27][40]. Moreover, an important role of cytotoxicity mediated by the death receptors FasL and TRAIL has also been observed
[41]. Time-lapse microscopy analyses indicate that a single NK cell can kill multiple tumor cells in a serial manner
[40][42]. In the serial killing of neoplastic cells susceptible to both death mechanisms, the granzyme-mediated death initially predominates, and the contribution of the pathway dependent on death receptors progressively increases
[40].
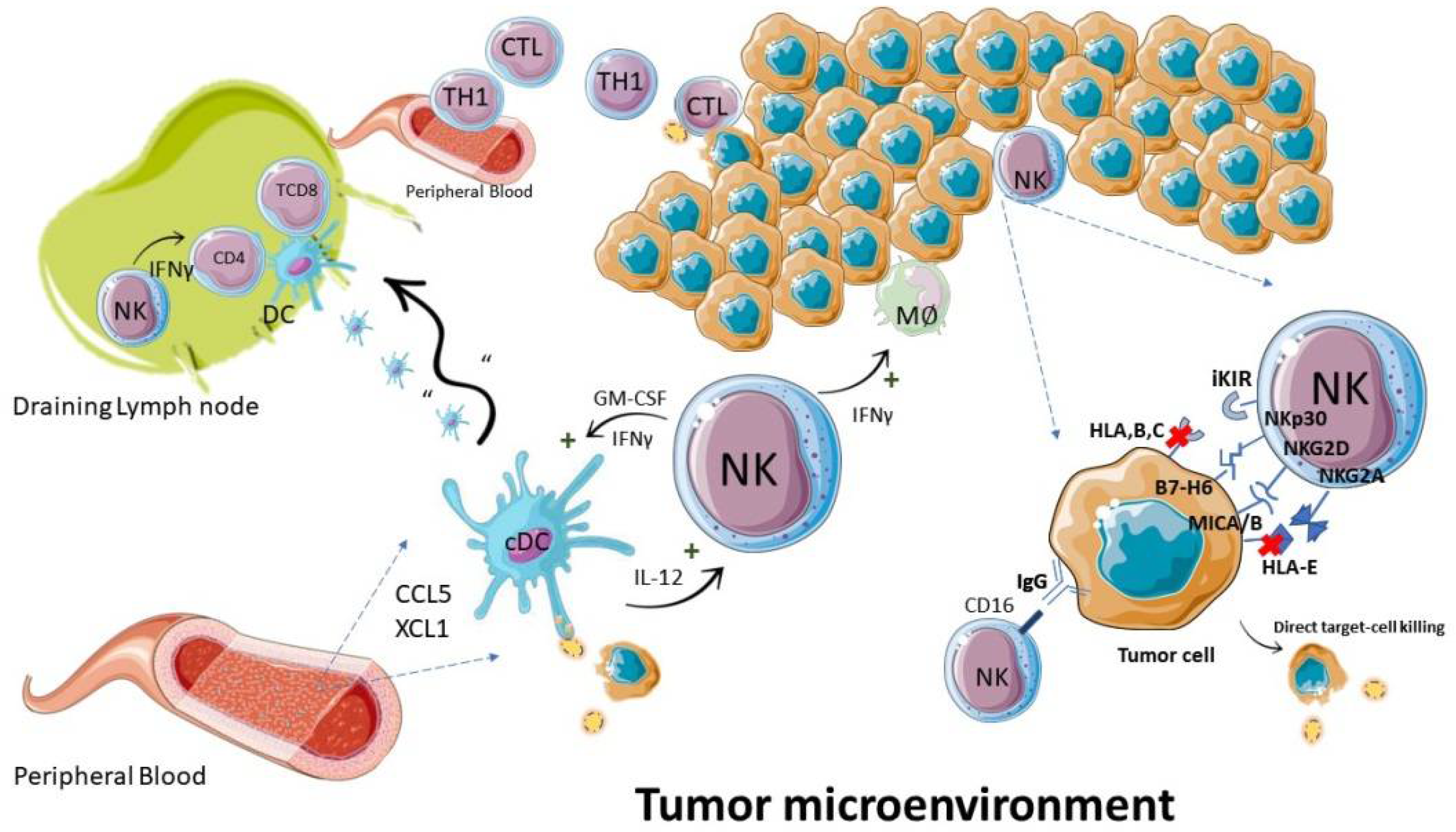
4. NK Cells in GBM
The dynamic blood–brain barrier (BBB) and other factors maintain a reversible state of immune privilege in the brain parenchyma, and prevent the entry of most peripheral blood immune cells into the non-inflamed central nervous system
[43]. However, glioblastoma cells disrupt astrocyte extensions involving blood vessels and modify vascular homeostasis, thus breaking the BBB
[44]. In addition, they maintain a pro-angiogenic and pro-inflammatory environment that leads to increased tissue permeability and chemotaxis. These changes support the recruitment of diverse immune cells into the tumor, which can be identified by immunohistochemical or flow cytometry techniques
[45][46][47][48][49]. It is also possible to obtain the sequences of the total RNA in GBM tissue stored in databases, and later use bioinformatics tools, such as CIBERSORT, to deconvolute immune populations according to the profiles of genetic signatures
[50][51].
In fact, many known mechanisms could counteract NK antitumor activities within the GBM. These include intrinsic characteristics of neoplastic cells and factors dependent on the tumor microenvironment, such as structural elements and the composition of the immune infiltrate
[52].
5. NK-Cell-Based Immunotherapy
Cancer immunotherapy has been an elusive goal ever since the proposition of the immunosurveillance hypothesis by Burnet
[53]. However, due to continuous elucidation of immune and tumor-evasion mechanisms, in recent years there has been a significant change in this previously dismal picture, and cancer immunotherapy has become a concrete option for many patients with various types of cancer
[54].
Due to the many roles that NK cells can play against cancer, as well as their ability to quickly recognize and attack cancer cells, without the need for previous sensitization
[55], while also presenting limited reactivity against healthy tissues
[56]. NK-cell-based immunotherapy presents the possibility of targeting tumors that still lack well-defined antigens for specific response, as well as the possibility of using allogeneic products prepared in advance, and that may be administered in multiple patients without causing graft-versus-host disease (GvHD)
[57][58][59], thus potentially leading to less toxicity in comparison to CAR T-cell infusions, for example
[56][59].
Different strategies of NK-cell-based immunotherapy for GBM are summarized in
Figure 3; they pursue different strategies, aiming at the number or in vivo persistence of these cells
[60], the activation or blocking of their inhibitory signals, their cytotoxic potential through adoptive cell therapies
[55], or sensitizing cancer cells to NK-cell-mediated lysis
[61].
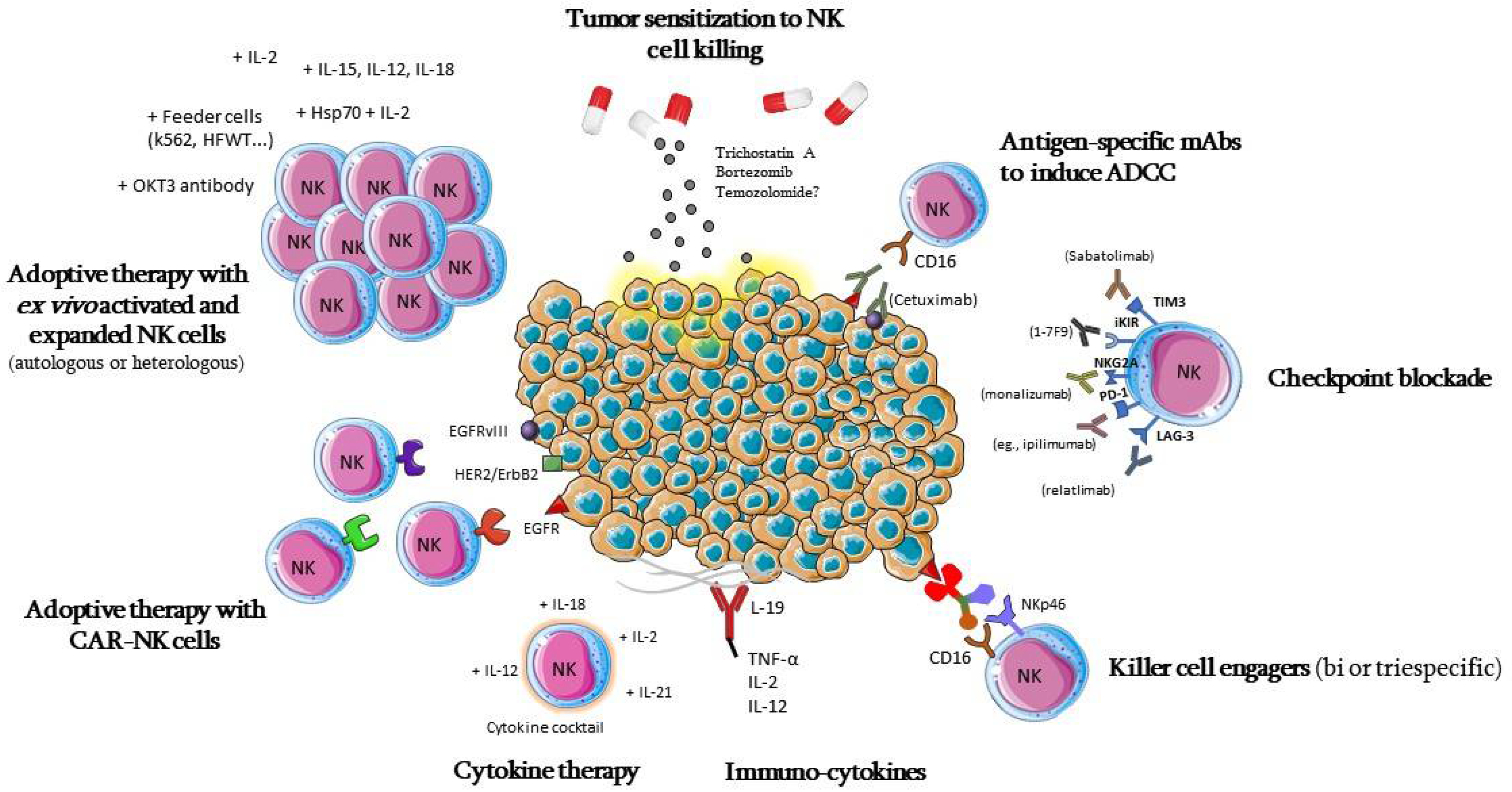
5.1. Adoptive Cell Therapy
Adoptive cell transfer (ACT) with NK cells is a strategy that involves the infusion of expanded and activated NK cells to have them act against the tumor. Currently, NK cells can be obtained at large scale through numerous methods. They can be isolated from the peripheral blood of patients, or from healthy donors (either from the postpartum placenta, the umbilical cord blood, or peripheral blood)
[56]. Other approaches involve the differentiation of NK cells from stem cells—such as induced pluripotent stem cells (iPSCs), human embryonic stem cells (hESCs), or hematopoietic stem cells
[56][62][63]—or the use of established human cell lines, such as the NK-92 cell line, averting the need for isolation and differentiation steps
[64].
5.2. CAR-NK Cells
The success of chimeric antigen receptor (CAR) T-cell therapy against hematological tumors, with three therapies already approved for commercialization by the FDA, has prompted interest in improving the design of CARs, and in expanding this technique to other cell types, such as NK cells. CARs were initially developed to enable T cells to recognize and act against a given tumor antigen, bypassing the need for MHC presentation, which is necessary for recognition by the TCR
[65].
CARs are engineered molecules composed of four main components (Figure 4): one single-chain variable fragment (scFv), derived from a monoclonal antibody, which binds to the antigen of interest, conferring specificity; the hinge or spacer, which gives support and flexibility to the scFv; a transmembrane domain; and one or more domains of intracellular signaling, responsible for cell activation [206].
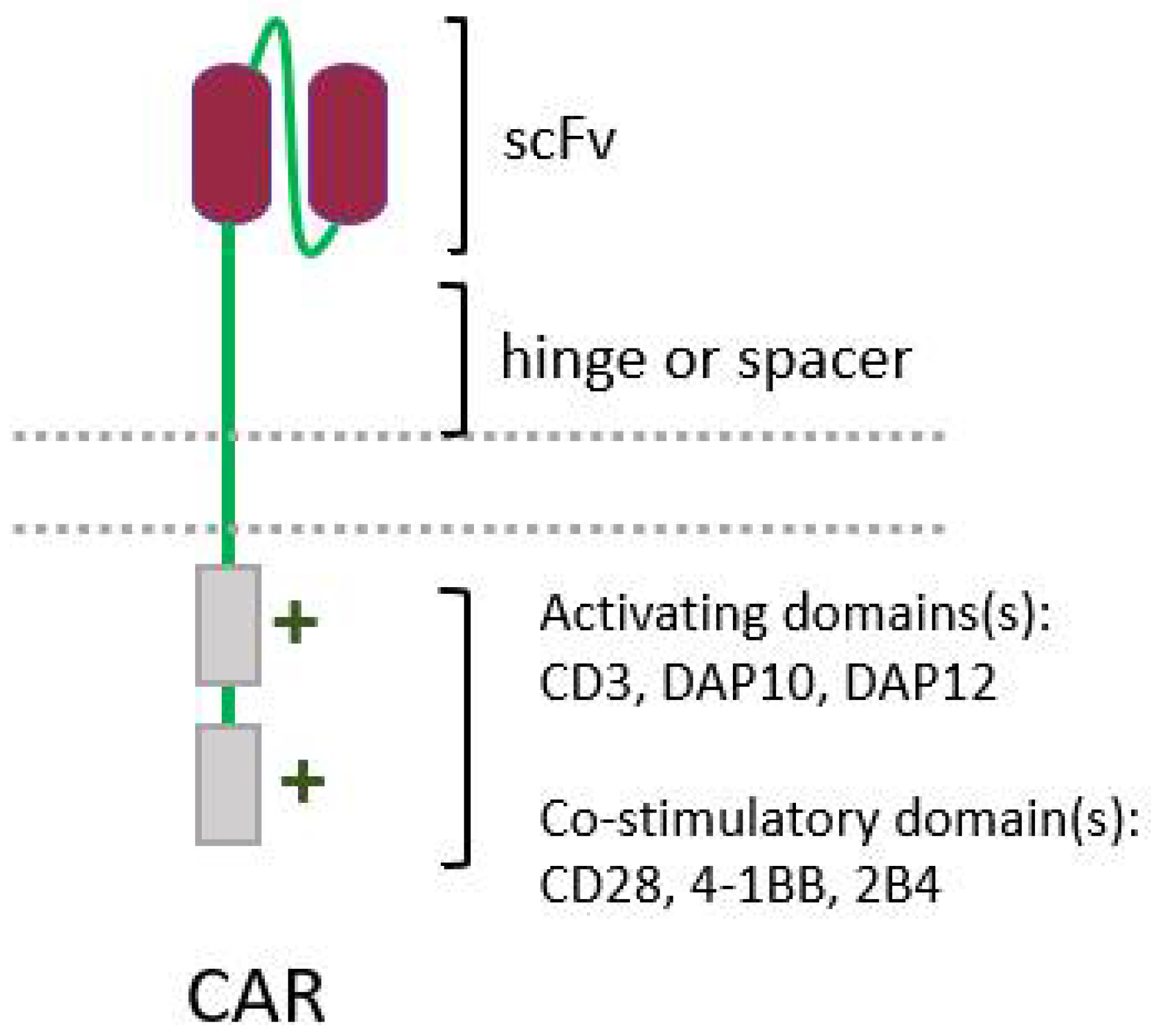
5.3. Cytokine Therapy
Cytokines influence all aspects of the immune response, and several have the potential to improve the function, proliferation, and survival of NK cells, and therefore can be used in NK-based immunotherapy, either by systemic administration or by in vitro incubation with NK cells in the process of generating the adoptive cells. IL-2, IL-12, and IL-15 are the main cytokines being explored in this context.
5.4. Monoclonal Antibodies, Killer Cell Engagers, Immunocytokines, and Other Antibody-Based Strategies to Boost NK Activation
A broad range of antibody-based therapies have been tested and approved for the treatment of various cancers. Since some of them can trigger NK cell activation, they can be used to overcome the dysfunction and immunosuppressed status of the patients’ NK cells, or even to improve adoptive cell therapy efficiency. One of that strategies consists of the use of monoclonal antibodies (mAbs) targeting tumor cells, rendering them susceptible to ADCC—a process that can be mediated by NK cells
[66].
5.5. Tumor Sensitization
The efficacy of adoptive therapies might be increased by the previous sensitization of the GBM cells to the cytotoxicity mediated by the NK cells. The proteasome inhibitor bortezomib (BTZ), which is an antineoplastic agent already approved and commercialized for the treatment of multiple myeloma and mantle-cell lymphoma
[67], is an eligible candidate for this function; data regarding its relation to the anti-GBM activity of NK cells have been reported recently by Navarro et al. (2019)
[61] and Luna et al. (2019)
[68]; both studies showed that primary GBM cell lines, when treated with BTZ, had a significantly increased membrane expression of ligands for the NK-activating receptor NKG2D, and also for the death receptor TRAIL-R2.
6. Conclusions
Despite the constant efforts over the past decades, GBM remains a challenging disease, and the prognosis of patients is still poor. Key characteristics of GBM—such as its intrinsic heterogeneity and the strategies it displays to overcome the immune system, which include several mechanisms of NK cell immunosuppression—make it partially resistant to the various antineoplastic treatments employed.
NK cells are lymphocytes of the innate immune system with particularities that could make them advantageous in antitumor responses: their natural cytotoxicity against neoplastic cells, their numerous helper functions and, consequently, their important contribution to immunosurveillance, and to response against established tumors. In agreement with this, NK cell immunotherapy has proven to be a powerful tool for the treatment of various experimental tumors, and initial clinical data from studies in GBM indicate a good safety profile and effectiveness for NK-cell-based immunotherapy.
The arsenal of strategies involving NK cells against GBM cells is certainly not limited to those mentioned here, since an increasing number of studies have been showing promising results, and the therapeutic possibilities are being radically expanded. Future strategies will benefit from screening for biomarkers associated with therapeutic efficacy, the combination of multiple therapeutic approaches and, ideally, personalized treatment regimens, considering the unique features of each patient. Thus, even if the contribution of NK cells for antitumor immune response in GBM patients still requires further study, they definitely appear to be a very promising and interesting component of the immune system’s response against GBM, which could be harnessed to improve treatment and, thus, patient care.