Microbiota-derived short-chain fatty acids (SCFAs), namely acetate, butyrate, and propionate, have the ability to modulate the tumor microenvironment in distinct ways. SCFAs promote immune cell differentiation, downregulate the expression of pro-inflammatory mediators, and restrict the tumor-induced angiogenesis. SCFAs also sustain the integrity of basement membranes and modulate the intestinal pH. Colorectal cancer (CRC) patients have lower concentrations of SCFAs than healthy individuals. Increasing the production of SCFAs through the manipulation of the gut microbiota could constitute an important therapeutic strategy towards CRC due to their antitumorigenic effect and ability of modulating tumor microenvironment.
1. Colorectal Cancer Therapeutic Challenges
Colorectal cancer (CRC) is one of the most common malignant tumors worldwide being the third in terms of incidence but the second in terms of mortality
[1]. Its incidence is related with lifestyle factors, such as a low-fibre diet, smoking, lack of exercise, and obesity
[2][3]. Despite the evolution on the research for new therapeutics, the management of CRC still poses several therapeutic challenges. Some of these challenges include late diagnosis since CRC often presents with no symptoms in its early stages making it difficult to diagnose until it has progressed to later stages, which can reduce the effectiveness of the treatment. The resistance to chemotherapy also constitutes a difficulty for an effective treatment, along with the high probability for the development of a metastatic disease. Importantly, the side effects associated with CRC treatments can impact the quality of life of patients
[4].
Despite all these challenges, there are several therapeutic options currently being clinically used to treat CRC. However, it is important to understand that CRC comprises two different types of tumors, namely colon cancer and rectal cancer
[5]. In fact, they are often grouped together due to the several common symptoms (such as bleeding, pain, and changes in stool), but anatomically the colon is approximately five feet long in the large intestine, while the rectum is the last five to six inches of the colon that connects to the anus
[5]. Taking this into account, depending on the diagnosis and stage of the disease, the first line of treatment for both colon and rectal cancers consists of achieving the complete removal of the tumor and metastases by surgical intervention
[6]. However, when the cancer is diagnosed at an advanced stage with metastases, the surgical control becomes difficult and, for those patients, the best option is to shrink the tumor, inhibiting the tumor spread and growth by chemotherapy
[6]. This strategy might also be applied before or after surgery as adjuvant treatment to maximally reduce and stabilize the tumor
[6].
2. The Tumor Microenvironment
The tumor microenvironment is a complex and continuously evolving entity, which consists of tumor cells, tumor stromal cells, including stromal fibroblasts, endothelial cells (ECs), and immune cells, and the non-cellular components of the extracellular matrix (ECM)
[7][8]. The interaction of the tumor cells with their microenvironment is dynamic and bidirectional and includes cell–cell contacts, or cell–free contacts (involving ECM), and the mediators that enable these contacts
[8]. The mediators can be soluble factors (chemokines, cytokines, and growth factors) or those that facilitate horizontal genetic/biomaterial transfer, namely cell-free DNA (cfDNA), apoptotic bodies, circulating tumor cells (CTCs), and exosomes
[8]. Through such complex crosstalks, the tumor microenvironment components are significantly involved in cancer progression and metastasis either by promoting or inhibiting the process
[8][9].
The microbiota is also an important element of the tumor microenvironment, and Hanahan suggests that polymorphic variation in microbiomes of the intestine and other organs, as well as the tumor microbiome, may constitute a distinctive enabling characteristic for the acquisition of hallmark capabilities
[10]. Microbiota play a dual role of promoting or inhibiting cancer progression, and its metabolites can be important modulators of the tumor microenvironment
[11].
The CRC tumor microenvironment, in particular, which includes the intestinal microbiota, is not only closely related to the growth and development of cancer but also affects the treatment and prognosis of the patients
[12]. It can limit the efficacy of therapeutic agents through high interstitial pressure, fibrosis, and the degradation of the therapeutic agents by enzymatic activity
[13]. Moreover, the tumor microenvironment is a special niche in terms of acidity, hypoxia, and ischemia, and its components can modulate tumor progression by stimulating angiogenesis, suppressing apoptosis, or inducing immunodepression
[13].
Due to the importance of the tumor microenvironment for the efficacy of the different available therapeutic agents, strategies for its modulation are being investigated in the cancer immunotherapy field. It is already known that the limited retention time is a problem for the approaches using drugs that target the tumor microenvironment. To overcome this limitation and allow the delivery of drugs in the tumor microenvironment, nanoparticles with unique physical properties and design have been developed
[14]. The possibility of creating an efficient drug delivery system with different ligands that could specifically target components in the tumor microenvironment, namely dendritic cells, macrophages, fibroblasts, tumor vasculature, and the hypoxic state, has been studied
[14]. These systems could also influence the aberrant structures and functions of the tumor microenvironment and in this way reducing the development of drug resistance and improving the response to chemotherapy. However, there are still several challenges to overcome in order to translate the “tumor-microenvironment-targeting nanoparticles” to clinical practice, one of them being the limited knowledge about the immune network of the different types of cancer, as well as the heterogeneity between the tumor microenvironment of different patients
[14].
3. Short-Chain Fatty Acids in the Human Colon
The intestinal microbiota, strongly modulated by the diet, plays an important role in maintaining host health, including protection against pathogens, maturation of the immune system, degradation of toxic substances, digestion of complex carbohydrates, and production of short-chain fatty acids (SCFAs)
[2]. SCFAs are mainly produced from dairy diet-derived microbiota, and they are the major products of bacterial fermentation of undigested dietary fibres and starch. They were shown to be able to influence the progress of several diseases, in particular inflammatory bowel disease (IBD), diabetes, atherosclerosis, and CRC
[15]. Gut microbiota-derived SCFAs, in particular acetate, butyrate, and propionate, can affect the energetic metabolism, enhance barrier function, reduce low-grade inflammation, and suppress tumor progression
[16]. The ratio of these metabolites in the colonic lumen is approximately 60% acetate, 25% propionate, and 15% butyrate, butyrate being the primary energy source for colonocytes
[2]. The level of SCFAs in faecal samples has been associated with some diseases, including cancer, being already reported that CRC patients have a decreased faecal SCFAs concentration compared to healthy individuals
[2][17].
Several studies have demonstrated the antitumorigenic properties of SCFAs
[18][19][20][21][22][23][24][25][26]. Among the three mentioned SCFAs, butyrate has been the most studied
[2]. It reduces survival and induces cell death in CRC cells through several mechanisms, depending on its intracellular concentration
[2]. Its protective effects against human colon cancer cells involve inhibition of cell differentiation, promotion of cell-cycle arrest and apoptosis, modulation of histone acetylation, and decrease of pro-inflammatory factors with an increase in the anti-inflammatory cytokine interleukin 10 (IL-10)
[2].
Propionate induces typical signs of apoptosis in human CRC cell lines with a loss of mitochondrial membrane potential, generation of reactive oxygen species (ROS), cytochrome c release, caspase-3-processing, and nuclear chromatin condensation
[2]. It can induce autophagy, which serves as an adaptive strategy to retard mitochondria-mediated cell death in CRC cells
[2]. Propionate, like butyrate, also acts as an inhibitor of cell growth and as an inducer of acetylation in CRC cells
[2].
Despite being the least studied, acetate also affects CRC cells
[2]. It was shown to decrease viability and to induce typical signs of apoptosis, including loss of mitochondrial membrane potential, generation of ROS, caspase-3 processing, and nuclear chromatin condensation in the colon adenocarcinoma cell line HT-29
[2].
4. Short-Chain Fatty Acids and the Tumor Microenvironment
4.1. Role of Short-Chain Fatty Acids in the Regulation of Immune Cells
Immune cells are critical components of the tumor microenvironment that can act both as suppressors of tumor initiation and progression, as well as promoters of proliferation, infiltration, and metastasis
[27]. Broadly, they fall into two categories: adaptive immune cells, such as T and B lymphocytes, and innate immune cells, including macrophages, mast cells, neutrophils, dendritic cells (DCs), myeloid derived suppressor cells (MDSCs), and natural killer (NK) cells
[10]. Both pro- and antitumorigenic roles of each cell type in the tumor microenvironment and treatment responses have been thoroughly described
[7][8][9][12][27]. A good prognosis in CRC has been attributed to infiltration by Th1 cells, M1 macrophages, mature DCs, and NK cells, while the presence of M2 macrophages, MDSCs, Th17, and B cells has been associated with a poor outcome
[28]. The increase of SCFA levels affect immune cells in distinct ways, inducing several effects in different immune cells (as summarized in
Figure 1). In lymphocytes, there is an increased chromatin accessibility, histone acetylation, cytokine production, and T and B cells differentiation. In fact, it was already described that ILC3s, T cells, and B cells in the intestine are the primary targets of regulation by SCFAs. This happens because the levels of SCFAs are highest in the gut, where SCFAs support the activity of these lymphocytes to promote balanced intestinal immunity and immune tolerance
[29].
Figure 1. The effects of short-chain fatty acids in the tumor microenvironment-associated immune cells.
Macrophage differentiation is also induced with associated anti-inflammatory effects. It was recently reported that butyrate directs the differentiation of homeostatic macrophages that possess strong antimicrobial activity through the inhibition of HDAC3 by regulating their metabolic and transcriptional program
[30].
SCFAs also promote anti-inflammatory effects through the inhibition of IL-6 and IL-12, the induction of IL-10 and IL-16 in dendritic cells, and consequent Treg differentiation. It was reported that the dendritic cells’ expression of amphiregulin, a molecule of the epidermal growth factor (EGF) family, which is a critical regulator of cell proliferation and tissue repair, depends on butyrate through its interaction with GPR43
[31]. Comparing the three metabolites, acetate only exerts negligible effects, while both butyrate and propionate are described as being able to strongly modulate gene expression in both immature and mature human monocyte-derived dendritic cells
[32].
Regarding neutrophils, these cells are attracted to the tumor microenvironment in response to SCFA treatment, consequently suffering apoptosis. A recent study demonstrated for the first time that SCFAs induce the formation of neutrophil extracellular traps (NET) when neutrophils are exposed to the intestinal physiological concentrations of these acids
[33]. The process of NET formation involves the release of chromatin structures in the form of an extracellular network containing nuclear or mitochondrial DNA with the objective of using these structures being to trap pathogens and prevent their spread in the organism
[33].
4.1.1. Effect of Short-Chain Fatty Acids in Lymphocyte Populations
Within the tumor microenvironment there are several distinct populations of lymphocytes
[7]. Cytotoxic T cells (CD8+) detect abnormal tumor antigens expressed on cancer cells and target them for destruction
[7]. They also suppress angiogenesis through the secretion of interferon gamma (IFN-γ)
[7]. CD4+ T cells differentiate into a variety of subtypes, including helper T cells, and thus coordinate a wide range of immune responses within the context of the tumor microenvironment
[7]. Regulatory T cells (Tregs) are ubiquitous and promote tumor development and progression by dampening antitumor immune responses
[7]. Additionally, Tregs directly support the survival of cancer cells through the secretion of growth factors and indirectly through interaction with stromal cells
[7]. B cells recognize tumor antigens and produce specific antibodies against the tumor with the cooperation of helper T cells, decreasing tumor progression
[34].
Other studies have suggested that butyrate can affect the tumor immune response via promoting T cell differentiation (
Figure 1) into effector and Treg cells
[35]. In many cancer therapies, less infiltration or dysfunction of CD8+ T cells in the tumor microenvironment results in poor clinical outcomes
[36]. Butyrate can modulate antitumor CD8+ T cell responses through the inhibition of differentiation 2-dependent IL-12 signaling, suggesting that this SCFA can promote anticancer immunity to sufficiently improve therapeutic efficacy
[36]. Most of the studies were conducted in mice, and whether butyrate could also influence antitumor CD8+ T cell immunity in humans remains to be determined, as well as whether different doses of butyrate could differentially regulate many cells in the tumor microenvironment. Additionally, butyrate downregulates CRC-related adverse events of indoleamine 2,3-dioxygenase 1 (IDO1) expression via a signal transducer and activator of a transcription 1 (STAT1)-dependent way or as the histone deacetylase (HDAC) inhibitor
[16]. IDO1 can activate β-catenin signaling to promote cancer cell proliferation and colon tumorigenesis
[16].
B cells, or B lymphocytes, participate in immune regulation mainly by immunoglobulin production, antigen presentation, and secretion of cytokines
[12]. Typically, they are concentrated at the margin of the tumors and they are commonly found in lymph nodes near the tumor microenvironment
[7]. Compared to T cells, relatively few infiltrating B cells are found in the tumor microenvironment, but they are important in the formation of “tertiary lymphoid structures” that allow close association between T and B cells
[7]. The antitumorigenic roles of B cells include antigen-presentation to T cells, antitumor antibody production, and secretion of cytokines, such as IFN-γ, which promote cytotoxic immune responses
[7].
4.1.2. Short-Chain Fatty Acids Modulate Macrophages
Macrophages are critical elements of the innate immune system, modulating immune responses through pathogen phagocytosis and antigen presentation, and are also critical in wound healing and tissue repair
[7]. In most types of solid cancers, the infiltration of tumor-associated macrophages (TAMs) is usually linked with a poor survival and enhanced metastasis
[37]. However, in CRC the infiltration of TAMs is linked with better prognosis
[37]. TAMs can be subdivided into two categories based on their activation status, M1 (classically activated) or M2 (alternatively activated), which in most cases are considered pro-tumorigenic
[37]. M1 TAMs are driven by IFN-γ, whereas alternative M2 TAMs are driven by IL-4 and IL-13
[37]. The tumor microenvironment promotes the M2 phenotype through hypoxia and the secretion of cytokines to support tumor growth and progression
[7]. M2 TAMs produce high levels of reactive oxygen free radicals, promote DNA damage and genomic instability, tumor infiltration and metastasis, participate in the digestion and reconstruction of ECM, and inhibit antitumor immunity
[38]. M2 TAMs marker expression is a poor prognostic factor in CRC
[34].
4.1.3. Dendritic Cells Modulation by Short-Chain Fatty Acids
Dendritic cells (DCs) are considered the most professional antigen presenting cells (APCs)
[37]. They recognize, capture, and present antigens to T cells at secondary lymphoid organs, linking the gap between adaptive and innate immunity
[7]. Ideally, DCs within the tumor microenvironment surround tumor associated antigens and migrate towards the draining lymph nodes, where they stimulate T cell-mediated responses
[37]. DCs are inherently programmed to have an antitumorigenic function in the body, but the tumor microenvironment can co-opt them to support tumor progression
[7]. In a suppressive environment that hinders their maturation, they become tolerogenic or regulatory DCs, which promote tumor cell survival
[37].
4.1.4. Short-Chain Fatty Acids Impact on Neutrophil Functions
Neutrophils are effector cells of the innate immune system, accounting for 50–70% of human circulating leukocytes and provide the first line of defense against many pathogens
[7][12]. Like other immune cells, they can both promote and suppress tumor formation and progression
[9]. As a tumor begins to grow, neutrophils are recruited to the tumor microenvironment and promote inflammation through the release of cytokines and ROS that stimulate tumor cell apoptosis
[7]. Later in tumor development, neutrophils promote tumor growth through modification of the extracellular matrix, releasing vascular endothelial growth factor (VEGF) and producing matrix metalloprotease (MMP)-9 to stimulate angiogenesis and, ultimately, tumor progression and local invasion
[7].
4.2. Role of Short-Chain Fatty Acids in the Regulation of Endothelial Cells
Vascular endothelium is a thin monolayer of endothelial cells (ECs) that help to orchestrate the formation of blood vessels
[7]. It separates circulating blood from tissues, delivers water and nutrients, maintains metabolic homeostasis, carries immune cells, and participates in the formation of new blood vessels (angiogenesis)
[7]. Angiogenesis is crucial for cancer progression by supplying oxygen and nutrients while removing toxic metabolites and also provides a conduit for tumor cell dissemination and metastasis
[9]. Hypoxia-inducible factors initiate vessel sprouting by instructing ECs to secrete proangiogenic factors, such as platelet-derived growth factor (PDGF), epidermal growth factor (EGF), and vascular endothelial growth factor (VEGF)
[7]. Tumor associated ECs also produce growth factor receptors, such as VEGF and EGF receptors, to enhance angiogenesis
[9]. In an autocrine and paracrine process, VEGF stimulates the migration of ECs to form new blood vessel lumens, and then ECs secrete proteins to form new basement membranes
[7]. Blood vessels in the tumor microenvironment often fail to achieve the final stages of maturation and lack proper cell-to-cell connections, resulting in leaky vasculature and enabling cancer cells to transverse it
[7].
4.3. Impact of Short-Chain Fatty Acids on pH
As explained by the Warburg effect, tumors present a constitutive and persistent upregulation of glycolysis, which results in chronic acidification
[39]. To adapt and survive in adverse conditions, including local hypoxia and poor vasculature perfusion, cancer cells upregulate membrane pH regulators as a self-defensive strategy
[39]. This keeps an intracellular pH ranging from neutral to slightly alkaline due to an increased secretion of protons to the tumor microenvironment, leading to its acidification
[39]. The acidic tumor microenvironment has been associated with certain key features of cancer aggressiveness (
Figure 2), including invasion, evasion from the immune system, increased angiogenesis, and resistance to therapy, making it an attractive target for therapy
[39]. Additionally, a low extracellular pH also contributes to drug resistance
[39][40][41]. The acidic pH gradient generated between intra- and extracellular space affects the distribution and uptake of weak base chemotherapeutic drugs
[40]. These drugs become charged (ionized form), which compromises their transport across the plasma membrane and their further cytoplasmic accumulation leading to a lower cytotoxicity
[39].
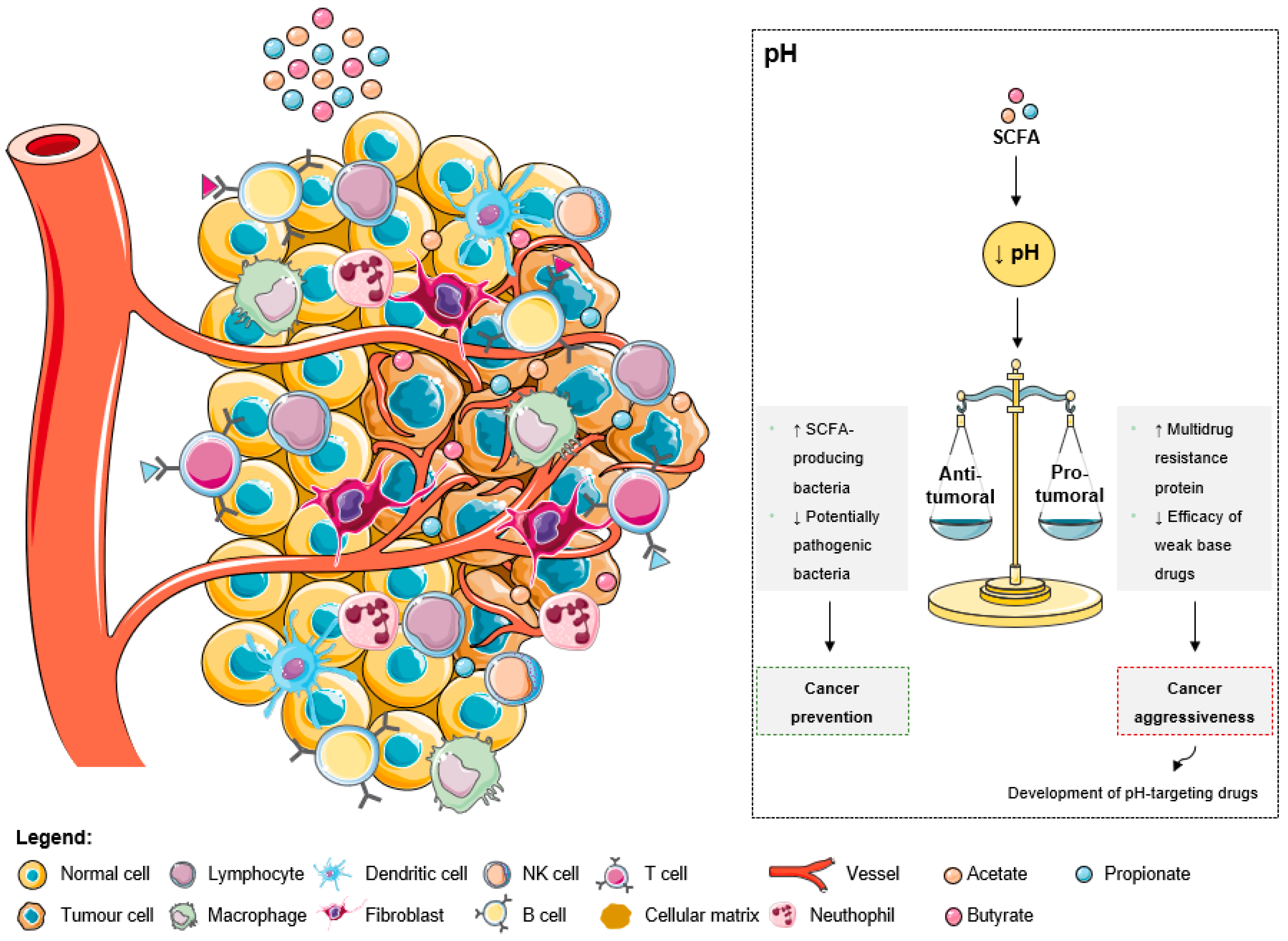
Figure 2. The effects of short-chain fatty acids in the tumor microenvironment pH. The increase of SCFA levels leads to a decrease of the extracellular pH. This acidic pattern contributes to cancer prevention due to the anti-tumoral effects, namely the increase of SCFA-producing bacteria and the decrease of pathogenic microbes. Additionally, the low extracellular pH could promote aggressiveness due to the increase of multidrug resistant proteins and the decrease of weak base drugs’ efficacy. However, these consequences are being overcome with the development of pH-targeting drugs.
4.4. Impact of Short-Chain Fatty Acids in Extracellular Matrix
The ECM is composed of glycoproteins, collagen, elastin, proteoglycans and other macromolecules, which support and connect tissues and maintain normal physiological functions
[12]. Compared with normal tissue, the ECM structure of tumor tissue is disordered
[12]. ECM proteins can be produced by many stromal cell types and tumor cells, however, cancer-associated fibroblasts (CAFs) are the main source for synthesis, secretion, assembly, and modification of the ECM composition and organization
[8].
Accumulation of significant amounts of collagen, together with a high percentage of fibroblast infiltration, result in desmoplasia, which is strongly linked to poor patient prognosis and resistance to therapy
[7][12]. In general, ECM abnormalities relieve the behavioural regulation of stromal cells and promote tumor-related angiogenesis and inflammation, resulting in resistance to immunotherapy in the tumor microenvironment
[12]. Each individual ECM protein contributes to CRC progression and metastasis in distinct ways, but the overall amount of ECM protein deposition contributes to the stiffness of the tumor microenvironment
[9]. Correspondingly, increased ECM stiffness is a hallmark of CRC progression and metastasis
[9].
5. Therapeutic Implications of the Tumor Microenvironment Modulation by Short-Chain Fatty Acids
Given the critical functions of the tumor microenvironment, from both microorganisms and the host, in CRC progression and metastasis and the accumulating knowledge on this subject, new insights about CRC therapy by targeting tumor microenvironment are emerging
[9]. One of the most common therapies for CRC is to target VEGF and prevent EC-mediated angiogenesis
[9]. However, this method has significant adverse side effects and limited benefit to the patients because it targets both tumor-associated and normal ECs
[9]. Aside from targeting or remodeling cellular components in the tumor microenvironment, manipulating non-cellular components could have therapeutic potential as well
[9].
Nowadays, research on a great variety of targets and approaches is being carried out
[12][42]. These include antiangiogenic therapy, adoptive cell therapy, immune checkpoint inhibitors, cancer vaccine and oncolytic virus therapy, and tumor-derived exosomes therapy
[12]. Several studies suggest that SCFAs can contribute to anticancer therapeutic efficacy
[43][44][45]. Approximately 50% of patients suffer from gastrointestinal mucositis after pelvic or abdominal radiation treatment, and the incidence is higher in patients undergoing concurrent chemotherapy
[43]. Besides the impact of gut microbiota on the response to diverse forms of cancer therapy, tumor treatments may in turn affect the microbiota (that is, induce dysbiosis), which can consequently aggravate the inflammation triggered by radiation and chemical reagents
[43]. SCFAs might contribute to restoring bacterial homeostasis, attenuating inflammation, maintaining the barrier function, promoting antitumor effects, and mucosal repair after cancer treatments
[43]. Furthermore, it was shown that the chemotherapeutical efficacy of 5-FU on CRC cells was promoted by the combined treatment of butyrate and 5-FU, with lower DNA synthesis efficiency and higher apoptotic cell ratios
[44]. It was also demonstrated that butyrate suppresses the proliferation of three-dimensional CRC organoids and enhances radiation-induced cell death in CRC organoids
[45]. However, butyrate does not increase radiation-induced cell death after irradiation in normal organoids
[45]. Accordingly, it may enhance the efficacy of radiotherapy while protecting the normal mucosa
[45]. These findings support the idea that adjusting food intake, regulating gut bacteria, and subsequently altering the concentration of SCFAs is a promising approach in CRC treatment.
This entry is adapted from the peer-reviewed paper 10.3390/ijms24065069