1. Modification and Doping of Graphene
Graphene monolayer is not inherently a highly conductive electrode material, because its defects R
s is about 1000 Ω/sq, and the lowest figure of merit (FOM) in the photovoltaic field up to date
[1]. As shown in
Figure 1a, ideal graphene provides a flawless transport pathway for electrons or holes, avoids the concern of high resistance losses when applied in organic optoelectronic devices. However, graphene monolayer is often produced with defects in the preparation process, as shown in
Figure 1b, graphene with defects affects its conductive properties as TE materials, there is a natural effect on the electronic structure, which leads to electron or hole transport is hampered and reduces the lateral conductivity of graphene, and results in low carrier concentration and a large potential energy barrier
[2]. Graphene and any other 2-d materials has a very low transmittance and is also a major reason why it’s used in conjugation with other materials and not standalone. Therefore, the modification and stable doping of graphene are extremely important to obtain graphene composite TEs with R
s below 100 Ω/sq and above 90% optical transmittance.
Figure 1. The schematic illustration of electron or hole transport path in: (a) ideal graphene provides a flawless transport pathway for electrons or holes, while it is hampered in (b) defective graphene.
2. Reduced Graphene Oxide
A common modification method for graphene is reduced graphene oxide (RGO), a low-cost method compatible with roll-to-roll (R2R) mass production, where graphene oxide (GO) is chemically peeled by ultrasonic dispersion or rapid thermal expansion, and then reduced by appropriate chemical or laser-assisted methods
[3]. RGO can be easily produced in large volumes as graphene ink by taking advantage of its better solubility in common solutions
[4]. Based on this situation, RGO TEs have been extensively researched. Without sacrificing the optical transmittance, RGO cannot effectively reduce the resistance and roughness of the bottom layer, therefore, similar to graphene monolayer, RGO also need combine together with other electrode materials
[5][6][7].
3. Composite Preparation of Graphene Transparent Electrodes
The conductivity of graphene monolayer is poor, in order to meet the application of graphene TEs in optoelectronic devices, as shown in
Figure 2, graphene monolayer is usually combined together with metal oxide, CNT, MNWs and metal thin film
[8][9][10][11].
Figure 2. The schematic illustration of graphene composite preparation with typical nanomaterials. Such as metal oxide, MNWs, CNT and metal thin film, resolved the poor lateral conductivity of graphene monolayer.
CNT films have excellent chemical stability and conductivity. However, the adhesion to the substrate is weak, and its unique tube-tube junction can easily open causing a rise in R
s, so CNT is often hybrid with other electrode materials such as graphene to prepare electrodes. On the basis of this, Geng et al. performed polydopamine (PDA) functionalized of the RGO as plotted in
Figure 3a, and thus combined with single-walled CNT (SWCNT)
[12]. As illustrated in
Figure 3b,c, PDA-RGO/SWCNT/poly(3,4-ethylenedioxythiophene) polystyrene sulfonate (PEDOT:PSS) composite TEs with a structure similar to that of reinforced concrete were fabricated. This structure has excellent mechanical stability during bending, and the PDA-RGO improves the adhesion of the conductive layer with the substrate. The conductivity of the composite electrode is significantly improved, with a sheet resistance of 52.2 Ω/sq and an optical transmission of 88.7% at 550 nm. The composite TE has the potential as an organic light-emitting diode (OLED) anode, and the prepared device has a luminance of 2032 cd/cm
2 at 15 V and maximum current efficiency of 2.13 cd/A at 14 V.
Figure 3. The schematic illustration of TCFs with ultra-adhesion, relatively low roughness, and excellent electrical conductivity: (
a) PDA−RGO/CNT and (
b) PDA−RGO/CNT/PEDOT:PSS. (
c) A picture of reinforced concrete structure used in building construction. (Reproduced from
[12] with permission from Elsevier, 2020).
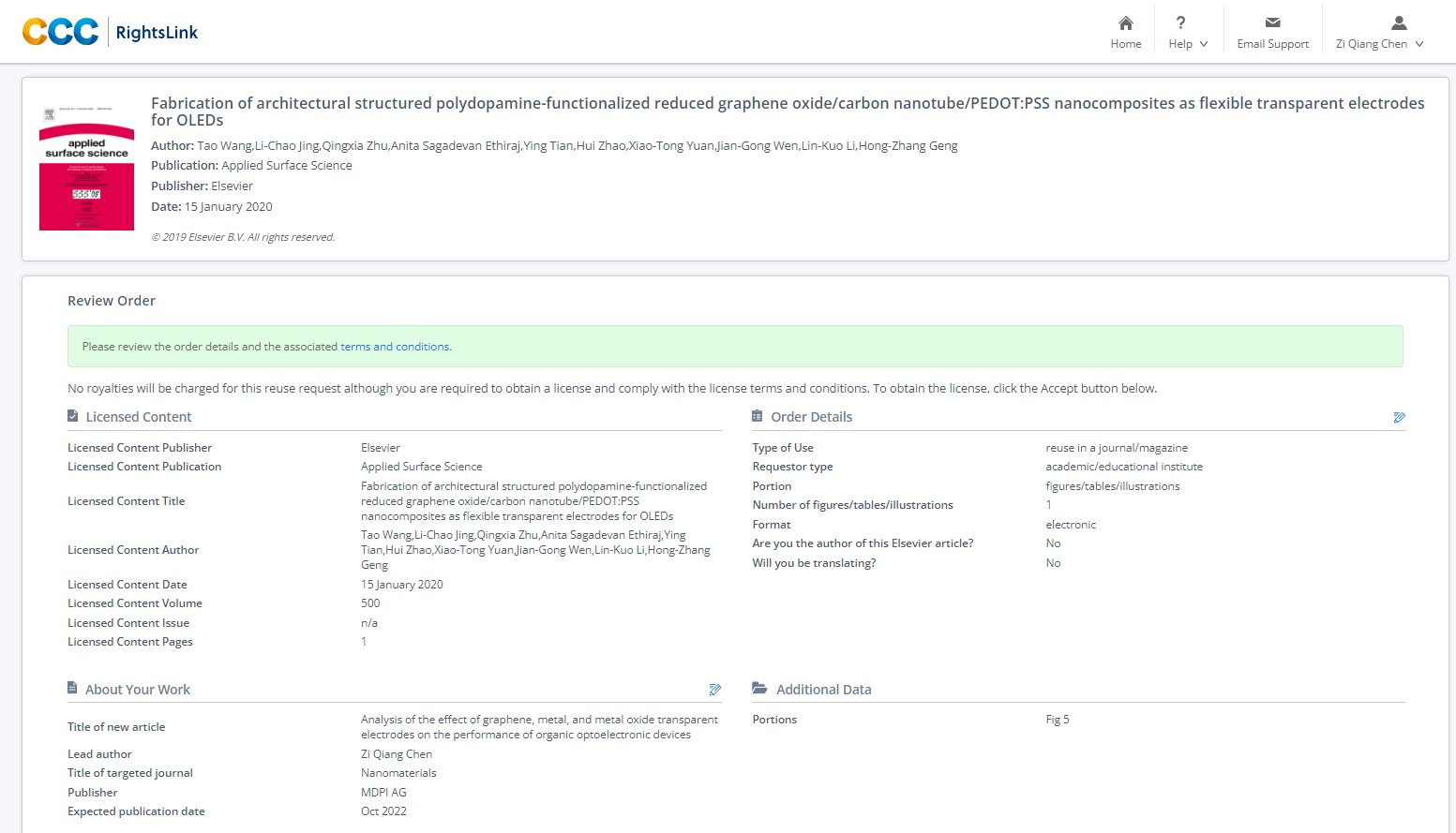
In the previous work, researchers provided a graphene optimization method, researchers prepared Au-doped graphene films, and through improving the concentration of dopant solution (AuCl
3) and doping time, researchers prepared flexible transparent graphene electrode with R
s reduced from 365 Ω/sq to 90 Ω/sq, and 85.6% transmittance at 550 nm. When applied in OLED device, it exhibited higher performance both in terms of illumination and device efficiency
[13]. MNWs are widely applied as TE materials
[14]. The distribution of MNWs is random and the conductivity is severely affected by boundary effects, which arise from the fact that the atoms on the surface of MNWs that are not fully bonded as those of the monolithic material, which makes the electrical conductivity of MNWs lower than that of the monolithic material
[15][16]. As the size of MNWs decreases, the number of surface atoms increases relative to the number of monolithic atoms, and thus the boundary effects become more pronounced
[17]. Good electrical conductivity requires upgrading the length of MNWs. However, micron-scale lengths can make the prepared films have significant protrusions, so MNWs are often hybrid with other electrode materials such as graphene to prepare electrodes.
Accordingly, Grossman et al. reported a highly stable electrophoretic deposition (EPD)-GO/silver nanowires (AgNWs)/GO TE, which has a similar sandwich structure fabricated by utilizing the EPD method for GO, where AgNWs serve as a conductive bridge covered by GO films on both sides
[18]. This newly developed all-solution process allowed transparent conductive films to be transferred to alternate surfaces after deposition and demonstrates excellent R
s (15 Ω/sq). In addition, simply changing the thickness of EPD-GO, its transmittance can be adjusted from 70% and 87% at 550 nm. In contrast with bare AgNWs, the composite electrode retains its original conductivity over long periods of operation at 80% relative humidity. the AgNWs networks are effectively “interposed” between the two layers of GO providing combined stability and performance.
In a research work published by Berry’s research team, it was demonstrated that ideally flawless graphene can be utilized as a multifunctional encapsulating layer, because the gaps within the aromatic rings of carbon are nearly zero due to the overlapping electron clouds, preventing the penetration of gas molecules, even tiny helium molecules
[19].
Accordingly, Grossman et al. utilized ultrathin GO as an encapsulation layer to protect the AgNWs from the PEDOT:PSS-hole transport layer (HTL), thus not affecting the energy level alignment of the device
[20]. EPD-GO/AgNWs/GO as an organic solar cell (OSC) anode to substantially improve the lifetime of inverted translucent OSC, without additional encapsulation, the lifetime of the entire device is increased by a factor of five.
4. ALD-Oxide on Graphene
ALD-oxide (Atomic Layer Depostion-oxide) is an essential material for the perovskite solar cell (PSC) electron transport layer, organic optoelectronic device encapsulation layer, and TEs dielectric layer. However, due to the surface structure of graphene and the step-covering deposition of ALD, ALD oxides are difficult to deposit on graphene, so it is a challenging task to utilize graphene and ALD-oxide simultaneously
[21][22][23][24]. Accordingly, as shown in
Figure 4, people usually deposited a functional layer before depositing the ALD-oxide on the graphene, which can be a useful aid to the deposition of the ALD-oxide
[25]. Herein, researchers utilized ethylene glycol (EG) as a precursor to prepare a functional layer to activate the graphene surface and thus improve the deposition of ALD-ZnO films
[26]. The prepared EG-Graphene/ALD-ZnO composite films exhibit a significant reduction in R
s, higher transmittance, smooth and uniform surface, excellent bending ability and energy level matching with other device components. Lee et al. utilized NO
2 to provide nucleation sites for the deposition of ALD-Al
2O
3 on graphene to increase surface reactivity, promoting in the deposition of uniform Al
2O
3 layers on graphene
[27].
Figure 4. The solution for the deposition of oxides on flexible graphene substrate. The schematics, respectively, illustrate the flexible substrate with graphene, the deposition of a functional layer on graphene, and the fact that the oxide can be deposited well after the deposition of the functional layer.
5. Other Methods
Contrary to doping and modification, graphene monolayer can also be prepared without composite with other conductive materials. For instance, adhesion enhancement between the graphene monolayer and substrate compensates the defects of the graphene monolayer by graphene multilayer.
Graphene monolayer has poor adhesion to the substrate, and the graphene surface is easily contaminated by dust during the process of peeling off from the metal foil or transferring to the target substrate. These challenges make it difficult to utilize in industrial production. Koo et al. combined graphene film directly with polyimide (PI) to obtain TEs with high flexibility and thermal stability
[28]. As plotted in
Figure 5, the PI containing dual functionalities on CVD-grown graphene, which acts as both a carrier film and a substrate for the graphene electrode, exhibits an ultra-clean surface, along with an optical transmittance of over 92%, a sheet resistance of 83 Ω/sq. In addition, the direct integration of PI improves the durability of the graphene electrode by reducing the mechanical exfoliation process of the graphene. The power conversion efficiency (PCE) of OSC has obtained significant progress of 15.2%.
Figure 5. 200-nm-thin PI and 10-mm-thick PI were applied as a carrier film during graphene transfer and a substrate for the graphene electrode, respectively (Reproduced from
[28]with permission from Elsevier, 2020).
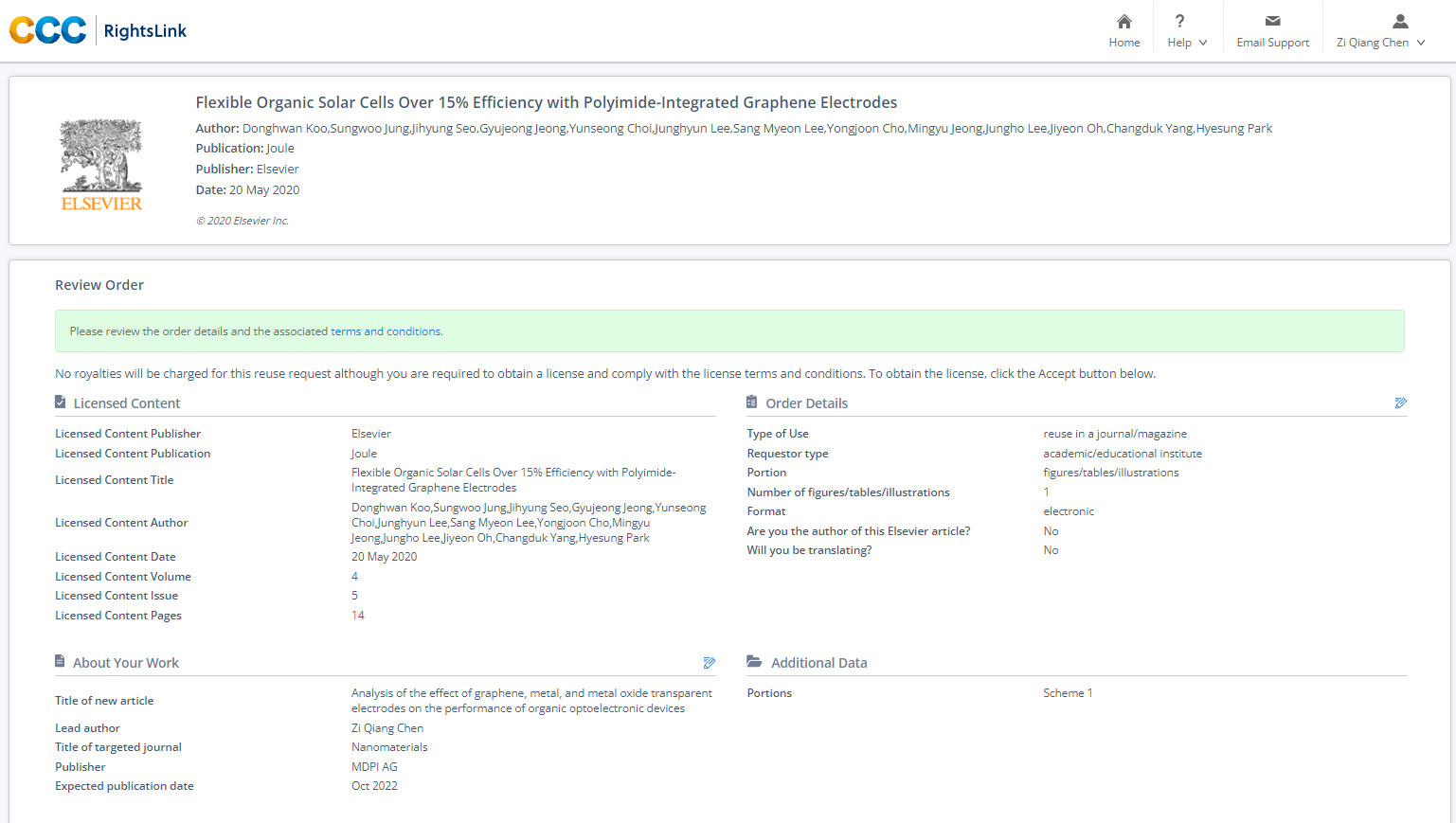
The photovoltaic properties of graphene multilayer, as compared to graphene monolayer, have also been extensively researched. However, contrary to the main theme of this review, therefore, it will not be described in detail.
This entry is adapted from the peer-reviewed paper 10.3390/nano13010025