Proteins play indispensable roles in maintaining cell survival, and their functions are often regulated by post-translational modifications (PTMs), in which proteins are proteolytically cleaved or enzymatically conjugated with modifying groups. Various enzymes, including kinases, phosphatases, transferases, and ligases, catalyze approximately 500 discrete PTMs of a diverse set of proteins. PTMs regulate diverse activities of a colossal number of proteins. For example, various types of lipids can be covalently linked to proteins enzymatically or non-enzymatically. Protein lipidation is perhaps not as extensively studied as protein phosphorylation, ubiquitination, or glycosylation although it is no less significant than these modifications. Evidence suggests that proteins can be attached by at least seven types of lipids, including fatty acids, lipoic acids, isoprenoids, sterols, phospholipids, glycosylphosphatidylinositol anchors, and lipid-derived electrophiles.
1. Introduction
Proteins play indispensable roles in maintaining cell survival, and their functions are often regulated by post-translational modifications (PTMs), in which proteins are proteolytically cleaved or enzymatically conjugated with modifying groups. Various enzymes, including kinases, phosphatases, transferases, and ligases, catalyze approximately 500 discrete PTMs of a diverse set of proteins [
1,
2]. PTM of proteins occurs at all stages of human life, and abnormal PTM often leads to various diseases [
3,
4,
5,
6,
7].
Well-studied PTMs include protein glycosylation, methylation, hydroxylation, amidation, phosphorylation, acetylation, and ubiquitination [
2,
8]. Protein lipidation is perhaps not as extensively studied as protein phosphorylation or acetylation even though it is no less significant than other modifications [
9,
10,
11,
12,
13]. Various lipids or lipid metabolites can be covalently attached to proteins, and this PTM is accordingly called under different names, including protein lipidation [
7], fatty acylation [
14], and lipid modifications of proteins [
15]. Nearly 20% of all proteins are known to be lipid modified [
16], which is relatively rare, resulting in the detection difficulty and need of enrichment techniques for characterization.
There exist many technologies to characterize lipidated proteins by taking full advantage of the proteins’ characteristics, including spectroscopic methods, such as nuclear magnetic resonance (NMR) spectroscopy [
17] and circular dichroism (CD) spectroscopy [
18] (according to membrane protein structure and dynamics), crystallography [
19,
20] (according to lipidated protein dimensional structure), mass spectrometry (MS) [
7] (according to lipidated protein fragment-ion characteristics), and so on. However, given the wide structural variability of lipid moieties of lipidated proteins, highly sensitive and specific methods for its detection are required [
21]. Adequate enrichment followed by MS will be a more effective strategy.
2. Types of Protein Lipidation
To date, studies have shown that proteins can be modified by at least seven types of lipids, including fatty acids, lipoic acids, isoprenoids, sterols, phospholipids, glycosylphosphatidylinositol (GPI) anchors, and lipid-derived electrophiles (LDEs).
2.1. Fatty Acylation
2.1.1. S-palmitoylation
Protein S-palmitoylation refers to the covalent attachment of palmitic acid (C16:0) to the side chain of a cysteine residue on a protein through a thioester bond (
Table 1). Palmitoylation is a reversible modification in organisms with specific enzymes to catalyze the addition or removal reaction [
22,
23], discovered in 1960s [
24,
25,
26,
27]. S-palmitoylation is dynamically regulated by palmitoyl acyltransferases (PATs, also known as ZDHHC-PATs) and acyl protein thioesterases (APTs), and the conserved zinc-finger Asp-His-His-Cys (ZDHHC) motif in the functional region of these enzymes is necessary for this process. PATs attach palmitoyl-CoA to proteins, and APTs perform depalmitoylation. For example, palmitic acid is added onto the SCRIB protein by the zinc finger DHHC-type palmitoyltransferase 7 (ZDHHC7) [
28] and removed by lysophospholipase 2 (LYPLA2, also known as APT2) [
29]. In some cases, however, proteins can be directly bound to palmitoyl-CoA and undergo PAT-independent auto-palmitoylation [
30]. To date, a family of 24 mammalian PATs has been identified [
7,
31]. The “SwissPalm” database shows that > 10% of the human proteome is susceptible to S-palmitoylation [
32,
33], in which >600 substrates have already been fully or partially characterized [
34,
35,
36]. Proteins that can be S-palmitoylated include NRas proto-oncogene, GTPase (NRAS) [
37], β
2 adrenergic receptors (β
2ARs) [
38], Fas cell surface death receptor (FAS) [
39], BCL2-associated X, apoptosis regulator (BAX) [
40], inositol 1,4,5-triphosphate receptor type I (ITPR1) [
41], junctional adhesion molecule 3 (JAM3) [
42], and SRC proto-oncogene, non-receptor tyrosine kinase (SRC) [
43].
Table 1. Types of cellular protein lipidation.
Modification
|
Lipid
|
Structure
|
Linkage
|
Modified Residue
|
References
|
1
|
S-palmitoylation
|
Palmitic acid (C16:0)
|

|
Thioester
|
Cysteine
|
[22,23,28,29]
|
2
|
N-terminal palmitoylation
|
Palmitic acid (C16:0)
|
Amide
|
N-terminal Cysteine
|
[31,44]
|
3
|
Nε-palmitoylation
|
Palmitic acid (C16:0)
|
Amide
|
Lysine
|
[45,46]
|
4
|
O-palmitoylation
|
Palmitic acid (C16:0)
|
Oxyester
|
Serine
|
[47]
|
Threonine
|
[48]
|
5
|
N-terminal myristoylation
|
Myristic acid (C14:0)
|

|
Amide
|
N-terminal Glycine
|
[49]
|
6
|
Nε-myristoylation
|
Myristic acid (C14:0)
|
Amide
|
Lysine
|
[50,51,52]
|
7
|
S-stearoylation
|
Stearic acid (C18:0)
|

|
Thioester
|
Cysteine
|
[53,54]
|
8
|
O-octanoylation
|
Octanoic acid (C8:0)
|
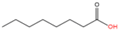
|
Oxyester
|
Serine
|
[55,56]
|
9
|
O-palmitoleoylation
|
Palmitoleic acid (C16:1n7)
|

|
Oxyester
|
Serine
|
[57,58,59]
|
10
|
N-oleoylation
|
Oleic acid (C18:1n9)
|

|
Amide
|
Lysine
|
[60]
|
11
|
Unnamed
|
Arachidonic acid (C20:4n6)
|

|
Yet unknown
|
Yet unknown
|
[61]
|
12
|
Unnamed
|
Eicosapentaenoic acid (C20:5n3)
|
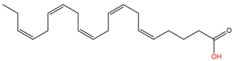
|
Yet unknown
|
Yet unknown
|
[61]
|
13
|
N-lipoylation
|
Lipoic acid
|
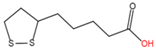
|
Amide
|
Lysine
|
[62,63]
|
14
|
S-prenylation
|
Isoprenoid
|

|
Untitled
|
C-terminal Cysteine
|
[64,65]
|

|
15
|
C-terminal phosphatidyl-ethanolaminylation
|
PE
|

|
Amide
|
C-terminal Glycine
|
[66,67]
|
16
|
C-terminal cholesterolyation
|
Cholesterol
|
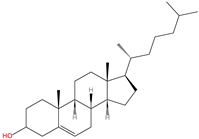
|
Oxyester
|
C-terminus
|
[68,69]
|
17
|
C-terminal GPI anchor
|
GPI
|
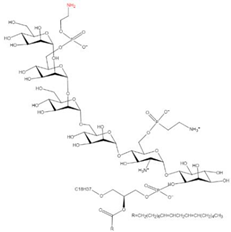
|
Amide
|
C-terminus
|
[70,71]
|
18
|
LDE acylation
|
LDE
|
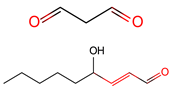
|
Carbonyls
|
Nucleophilic residues
|
[72,73]
|
Aldehydes
|
N-system nomenclature was used for the fatty acids (the order of carbon atoms starts from the methyl carbon of the fatty acid).
2.1.2. N-palmitoylation
N-palmitoylation is classified into N-terminal palmitoylation and N
ε-palmitoylation according to the position of the modification in the protein (
Table 1). In N-terminal palmitoylation, palmitic acid is linked to the amino group of the cysteine residue at the N-terminus of substrate proteins, whereas in N
ε-palmitoylation, palmitic acid is covalently attached to the ε-amino group of the lysine residue at the N-terminus via an amide bond. The biological significance of N-terminal palmitoylation has been reviewed before [
31,
44,
74]. A unique dual palmitoylation in the N-terminal region of the human LIM domain kinase 1 (LIMK1) controls the targeting of this protein to the spine and contributes to the activation of the protein by membrane-localized p21-activated kinase (PAK) [
74]. N-terminal palmitoylation has also been detected in Sonic Hedgehog (SHh) proteins [
75] and shown to be catalyzed by Hedgehog acyltransferase (HHAT) [
76]. Additionally, Sirtuin (SIRT) has been reported to be modified by N
ε-palmitoylation [
45,
46,
77,
78].
2.1.3. O-palmitoylation
Palmitic acid can be irreversibly linked to the side chain of serine residues in proteins via ester bonds in organisms without specific enzymes removing the attached lipid chain (
Table 1). Currently, only a few proteins are known to be O-palmitoylated. One of them is histone H4, which can be O-palmitoylated at Ser-45 by an enzyme called lysophosphatidylcholine acyltransfer ase 1 (LPCAT1) [
47]. Interestingly, O-palmitoylation at the threonine residue in the C-terminal of the spider venom neurotoxin PLTX-II has been reported, and it is thought to regulate the toxin activity in blocking presynaptic voltage-gated Ca
2+ channels [
48].
2.1.4. N-myristoylation
Similar to N-palmitoylation, N-myristoylation is also categorized into two major classes—N-terminal myristoylation and N
ε-myristoylation (
Table 1). N-terminal myristoylation is the attachment of myristic acid (C14:0) to a protein N-terminus with a glycine residue through an amide linkage. This reaction is catalyzed by N-myristoyltransferases (NMTs), which recognize the GXXXS/T signature sequence (where X is any amino acid) in substrate proteins [
49]. Human proteomic studies have suggested that > 100 proteins are N-myristoylated [
79], such as A-kinase anchoring protein 12 (AKAP12) [
77], SRC [
78], protein kinase AMP-activated non-catalytic subunit beta 1 (PRKAB1) [
80], FMR1 autosomal homolog 2 (FXR2) [
81], and hexokinase 1 variant in mammalian spermatozoa (HK1S) [
82], underscoring the vital roles of N-myristoylation [
83]. N
ε-myristoylation refers to the attachment of myristic acid to the ε-amino group of a lysine residue in substrate proteins. The enzyme(s) that catalyze this PTM is (are) not known; however, N
ε-myristoylated proteins can efficiently be deacylated by SIRTs [
50,
51,
52].
2.1.5. Acylation of Other Saturated Fatty Acids
In addition to palmitoylation and myristoylation, proteins can be lipidated with other types of long-, medium-, or short-chain saturated fatty acids (
Table 1). Studies have shown that influenza virus hemagglutinin can be S-acylated by stearate (C18:0) [
53,
54], Ghrelin can be O-octanoylated (C8:0) [
55,
56]. In 2009, the Zhao group released a bioinformatic tool named PTMap [
84], which helps identify various acylations, including Lys propionylation, butyrylation, hydroxyl-fatty acid modification, lactylation, bicarboxylic acid modification, and benzoylation. Numerous studies have discovered >500 histone modification sites for above listed modifications, greatly expanding the understanding of PTM and histone modifications [
85].
2.1.6. Acylation of Unsaturated Fatty Acids
Physeterylation (C14:1n9) is detected in the retina, heart, and liver [
86] and on SRC family kinases [
87]. Myristoleoyted (C14:1n5) proteins have also been found [
88,
89]. WNT proteins are O-palmitoleoylated with palmitoleic acid (C16:1n7) on their conserved serine residue by the O-acyltransferase Porcupine [
57,
58,
90], and the palmitoleic acid of an O-palmitoleoylated WNT protein is removed by Notum [
59]. Oleic acid (C18:1n9) modification has been reported on the lysine residue of the lens integral membrane protein aquaporin-0 and plays an important role in targeting the substrate protein to membrane domains in the bovine and human lens [
60] (
Table 1).
2.2. N-lipoylation
Lipoylation, the attachment of lipoic acid to a lysine residue in proteins (
Table 1), is a relatively rare PTM associated with human metabolic disorders, cancers, and mental diseases [
91]. The deacylase of lipoylated proteins is SIRT [
62,
63]. In mammals four multimeric metabolic enzymes—pyruvate dehydrogenase (PDH), α-ketoglutarate (KDH), branched-chain keto acid dehydrogenase E1 subunit alpha (BCKDHA), and glycine cleavage system (GCV)—are lipoylated and participate in the TCA cycle [
91,
92]. This modification confers a “swinging arm” conformation to the protein structure for enzymatic reactions [
91].
2.3. S-prenylation
S-prenylation is the attachment of isoprenoids to a cysteine residue in proteins [
65]. Up to 2% of the total cellular proteins in mammalian cells are prenylated [
93]. This modification occurs on one or more sidechains of a cysteine residue located at or near the C-terminus of the protein substrate. Most S-prenylated proteins contain a CAAX motif at their C-terminus, where the As are aliphatic amino acids and the X can be any amino acid [
64]. Based on the properties of the X residues, S-prenylation is categorized into two major types. If the X is a leucine or any other small residue (alanine/serine/methionine), a 20-carbon geranylgeranyl group is attached to the C-terminus of the protein substrate (i.e., S-geranylgeranylation). Otherwise, a 15-carbon farnesyl isoprenoid lipid is attached (i.e., S-farnesylation) [
65]. The enzyme that catalyzes protein S-farnesylation is called farnesyltransferase (FTase), whereas S-geranylgeranylation is catalyzed by geranylgeranyltransferase type I (GGTase-I) (
Table 1) or GGTase-II (also known as RabGGTase due to its specificity for Rab proteins) [
90]. Inhibitors of FTase and GGTase-I are used to target Ras prenylation, especially for KRas proto-oncogene, GTPase (KRAS), which is frequently mutated in many types of cancers [
94,
95].
2.4. C-terminal Phosphatidylethanolaminylation
C-terminal phosphatidylethanolaminylation is the attachment of phosphatidylethanolamine (PE) to the amino group of a C-terminal glycine (
Table 1). Microtubule-associated protein 1 light chain 3 alpha (LC3), a well-known protein associated with autophagy, is phosphatidylethanolaminylated [
66,
67].
2.5. C-terminal Cholesterolyation
C-terminal cholesterolyation is observed in Hedgehog (HH) family proteins and refers to the conjugation of cholesterol to the C-terminus of these proteins via an esterified linkage with the hydroxyl moiety of the cholesterol through an autocatalytic reaction (
Table 1). The HH family plays fundamental roles in long-range embryonic signal transduction pathways [
96]. HH proteins can undergo two types of modification, namely C-terminal cholesterolyation and N-terminal palmitoylation, which are both critical for the activities of HH proteins [
68,
69,
97].
2.6. C-terminal GPI Anchoring
Glycosylphosphatidylinositols (GPIs) are synthesized within the ER membrane through successive addition of a monosaccharide, acyl group, and phosphoethanolamine residue to phosphatidylinositol, consequently forming complex glycolipids. These glycolipids can be covalently attached to the C terminus of proteins by a GPI transamidase complex [
70] and removed by phosphatidylinositol-specific phospholipase C (PI-PLC) [
71] (
Table 1). Approximately 1% of eukaryotic proteins are GPI-anchored [
98] and participate in many biological processes, including cellular communication, signal transduction, antigen presentation, oncogenesis, malaria, and neurodegenerative prion diseases [
99,
100,
101,
102].
2.7. LDE Acylation
Lipid-derived electrophiles (LDEs) refer to the reactive lipid metabolites generated by lipid peroxidation or other metabolic pathways [
103]. Endogenous accumulation of oxidized lipid products has been reported as a biomarker of oxidative stress [
104]. LDEs include acrolein, malonaldehyde (MDA), 4-hydroxy-2-nonenal (4-HNE), 15-deoxy-D12, 14-prostaglandin J2 (14-PGJ2), and 2-
trans-hexadecenal (2-HD). These lipid metabolites can form covalent adducts with nucleophilic residues of proteins, such as cysteine, lysine, and histidine, via Michael addition (e.g., α, β-unsaturated carbonyls) or irreversible Schiff-base formation (e.g., aldehydes) [
72,
73] in organisms (
Table 1). More than 2300 proteins and 500 cysteine sites in cell lines have been reported to be targeted by acrolein [
105]. In a process called γ-oxononanoylation, 4-oxo-2-nonenal (4-ONE) attaches to the lysine residues of histones [
106], a modification that can be reversed by SIRT2 in organisms [
107].
3. Detection of Protein Lipidation
Detection of lipidated proteins involves challenging steps, including enrichment to identify the modification type and site, stoichiometric quantitation of the modification, and visualization of the modified protein. Despite these limitations, significant progress in the characterization of lipidated proteins has been made in the past few years.
The common “bottom up” high-throughput proteomics is considered a suitable approach to address these challenges through enrichment and digestion, multi-dimensional chromatographic separation, and high-throughput mass spectrometry detection [
108,
109,
110,
111]. Although MS-based detection approaches are highly sensitive in identifying lipidated proteins and modification sites, these approaches often require specialized protein enrichment methods, where is a filed hard to break through. Especially, for some very hydrophobic lipidated proteins, the common “bottom up” proteomics tends to underrepresent them. Thus, some studies have focused on detecting hydrophobic proteins with specific MS technique. Among them, the group of Robinson [
112], who created a new technology—gas-phase structural biology MS to study hydrophobic proteins and protein-lipid interactions [
113], while still deficient for high-throughput detection of lipidated proteins [
114].
4. Detection of PUFA-Modified Proteins
4.1. Difficulties in Detecting PUFA-Modified Proteins
Humans are estimated to express 20,000 proteins [
167,
168] (
https://www.hupo.org/human-proteome-project/, accessed on 2 February 2022), and PTMs of proteins play vital roles in diverse biological processes. Protein lipidation accounts for a small fraction of PTMs and of which PUFA modification represents the minority. Therefore, detection of the low levels of PUFA-modified proteins is challenging. Furthermore, double bonds in fatty acids are relatively unstable and PUFAs, especially ω-3 PUFA, are prone to peroxidation [
169,
170]. The peroxidation of PUFAs may happen in vivo as a part of the biological process or in vitro during sample enrichment processes in practice.
Saturated acyl chains can tightly pack with cholesterol to form ordered microdomains, such as membrane rafts, whereas unsaturated acyl chains do not pack well with cholesterol and thus form a disordered, liquid phase [
171,
172]. Unlike saturated fatty acids, PUFAs can target proteins to various microdomains and require diverse protein extraction procedures due to their structural complexity.
4.2. Limitations in Current Methodology
Concurrent accurate detection of abundant and scarce proteins via MS-based, high-throughput proteomic analyses is challenging [
173,
174,
175]. Although it is feasible to use the ABE method for the enrichment of PUFA-modified proteins, this method is specific for thioester-bond analysis, and PUFA modification of proteins through other bonds cannot be detected. It is important to distinguish proteins directly modified with LDEs from those initially modified by PUFAs and then oxidized. However, there is currently no method available for this purpose.
4.3. Potential Solutions
To characterize PUFA-modified proteins, a method that combines ABE and methyl esterification PUFA to GC/LC-MS detection and thereby detects lipidated proteins and simultaneously analyzes their fatty acid moieties is better [
150,
176,
177]. In this method, cells are washed with PBS and then lyzed with acetone. Afterward, proteins are precipitated, and the free fatty acid content of the supernatant is characterized (GC/LC-MS A). The protein pellet is suspended, and free thiol groups are blocked with NEM. The sample is then subjected to lipid extraction with chloroform/methanol, and the free fatty acid content of the upper layer is characterized (GC/LC-MS B). The proteins in the middle layer are resuspended, divided into two, and then treated with or without NH
2OH. The supernatant and pellet are used for the characterization of the fatty acids (GC/LC-MS C) and identification of the modified proteins (LC-MS D), respectively (
figure 1A).
figure 1. Methods to detect PUFA-modified proteins. (A) Flowchart of ABE and GC/LC-MS. Group A treats the supernatant from the acetone precipitation in cells; Group B treats the precipitation from the acetone precipitation in cells; Group C1 and D1 (+NH2OH group) add NH2OH and acetone to the above precipitation and further treats the second supernatant and precipitation as group C1 and D1; Group C2 and D2 (-NH2OH group) add control and acetone to the above precipitation and further treats the second supernatant and precipitation as group C2 and D2; (B) The synthesis of the alkynyl-linoleic acid (alk-LA) probe. (C) Flowchart of the Click-chemistry method employed on total-protein or membrane-protein samples.
The above ABE/GC-MS method is specific to S-fatty acylated proteins. To detect other potential PUFA-protein linkages, the researchers propose a synthesized alkynyl-linoleic acid (alk-LA) probe (
figure 1B) in light of the synthetic method of alkynyl-palmitic acid (alk-PA) probe [
178] and used the click-chemistry method for high-throughput detection of LA-modified proteins. In this strategy, the cells are incubated with the alk-LA probe for 24 h. Then, total proteins and membrane proteins are extracted, followed by the click-chemistry reaction. Afterward, the modified proteins are pulled down using streptavidin beads, digested, and finally analyzed via LC-MS (
figure 1C) [
140].
Another approach is the using of “top down” proteomics to identification lipidation by MS of intact proteins. A number of intact proteins recognition technique have emerged in recent years [
186], and further fueled by increase in biosimilars [
187]. Generally, increased peak capacity with advanced packing material as well as longer separation columns or integrating IMS significantly improves performance in “top down” and “bottom up” proteomics [
186,
188].
It is noteworthy that the alk-LA probe click chemistry method also effectively distinguishes PUFA-modification from LDE-modification since only LA-acylated proteins can be pulled down in this method. To minimize the peroxidation of the LA moiety of modified proteins, the samples should be supplemented with antioxidants.
In addition, whether PUFA-acylated proteins are tethered onto cellular membranes can be determined. For this purpose, the membrane fraction of the samples should be enriched first. PUFA-modified proteins may be concentrated by taking advantage of their double-bonded feature.
This entry is adapted from the peer-reviewed paper 10.3390/ijms23042365