1. Introduction
Nanoremediation technologies involve the use of reactive NPs for conversion and detoxification of contaminants. The main mechanisms for remediation by NPs are catalysis and chemical reduction
[1][2]. In addition, adsorption is another removal mechanism facilitated by the NPs since NPs have high surface-area-to-mass ratios and different distribution of active sites, increasing the adsorption ability
[3]. Many engineering NPs have highly feasible characteristics for in situ remediation applications due to their innovative surface coating and minute size. In addition, NPs can diffuse and penetrate the tiny spaces in the subsurface and be suspended in groundwater for a long time; compared to microparticles, NPs can potentially travel long distances and achieve larger spatial distribution
[1].
The physical movement of NPs and/or transport in groundwater is dominated by random motion or Brownian movement rather than the wall effect as a result of their nanoscale characteristics
[4]. Thus, compared to microscale particles, which are strongly influenced by gravity sedimentation due to their density and large size, the movement of NPs is not controlled by gravity sedimentation, remaining suspended in groundwater during the remediation process. Thus, NPs afford a functional treatment approach allowing direct injection into the subsurface where pollutants are present
[1].
Several studies have revealed the potential use of nanoremediation for soil and groundwater
[5][6][7][8]. However, the environmental effects of those NPs are still unclear and need more investigation to understand the environmental fate and toxicity of NPs, as these issues are crucial for environmental protection practice.
The use of nanomaterials for soil and groundwater remediation has been widely tested at the laboratory level against a large number of contaminants, offering promising results
[9][10]. However, nanomaterials may pose positive or negative impacts on living organisms, the environment, society, and the economy, which should be evaluated in a case-specific context. Appropriate documentation of nanoremediation risks, field-scale validation of remediation results, science–policy interface consultations, and suitable market development initiatives are ways to increase the popularity and acceptability of nanoremediation technologies
[11]. Savolainen et al.
[12] stated that the fundamental elements of risk assessment are likely to remain and will continue to include the elements designed for other chemicals and particles, notably (1) hazard identification, (2) hazard characterization, (3) exposure assessment, and (4) risk characterization, which are the four steps of the risk assessment process
[12]. However, the environmental effects of those NPs are still unclear and need more investigation to understand the environmental fate and toxicity of NPs as these issues are crucial for environmental protection practice
[13][14].
Various nanomaterials have been investigated for soil and groundwater remediation, such as metal oxides, nanoscale zeolites, enzymes, carbon nanotubes and fibers, titanium dioxide, and noble metals
[1]. Generally, zero-valent iron (nZVI) is most widely used for soil and groundwater remediation as nZVI is considered a suitable electron donor and highly reactive
[15]. The use of these different nanomaterials will be discussed in detail in this review.
2. Relationship between Soil and Groundwater: Contaminants and Remediation
Soil and groundwater are susceptible to pollution by a wide array of pollutants such as petroleum hydrocarbon, chlorinated solvents, and heavy metals.
[16]. Selecting a proper remediation technology for a contaminated environment usually depends on contaminant characteristics and contaminated site characteristics such as physical, chemical, and biological properties. All these factors should be considered during the remediation process, design, and implication. Moreover, the time/cost constraints, the regulatory requirements, and the remediation mechanisms should be considered in the selection process.
Nevertheless, adopting risk-based management approaches is increasingly a focus of environmental researchers due to the high demand for sustainable responses to environmental pollutions
[1][17]. The polluted environments are usually surface water, sediments, soil, and groundwater, which are mainly contaminated with low and high molecular weight petroleum hydrocarbon compounds, semi-volatile organic compounds, volatile organic compounds, polycyclic aromatic hydrocarbons (PAHs), polychlorinated biphenyls, persistent organic pollutants, organochlorinated pesticides, NAPL, hydrophobic organic compounds, heavy metals, and xenobiotics
[18][19][20]. These pollutants may migrate or spread far from the source and seriously affect flora, fauna, and the ecosystem
[17]. Managing the polluted environments requires the selection of the proper remediation technology for the pollutants, destruction, and separation methods according to many ex situ and in situ remediation methods for surface water, sediments, soil, and groundwater comprising physicochemical, biological, chemical, thermal, electromagnetic, electric, and ultrasonic remediation technologies
[21][22][23]. Remediation in an aqueous environment includes remediation of groundwater and surface water polluted by contaminants, whereas soil remediation includes remediation of sediment subsoil and topsoil polluted by contaminates
[24]. Soil and groundwater remediation could be conducted separately or together, depending on the concentration of contaminants and the extent of pollution. The efficiency of remediation technology depends on the design and implication based on the characteristics of polluted soil and the remediation technique. Combining one remediation technology with others sequentially or simultaneously may enhance the overall remediation process through combined or synergistic effects
[25].
3. Combined Nanoremediation with Other Remediation Technology
The combination of nanoremediation technologies with other mitigation methods has attracted significant research in recent years. Synergetic studies can be characterized as combining multiple nanoremediation methods simultaneously or combined with soil flushing or with biotreatment. In this section, an overview of the recent work in this domain is presented.
Several studies combined many nanoremediation methods at the same time. Vilardi et al.
[26] examined the combination of nZVI and CNTs for the remediation of Cr(VI), selenium (Se), and cobalt (Co) from aqueous solutions by conducted a batch experiment
[26]. The result indicated that for Cr(VI), the main removal mechanism the reduction, whereas adsorption was the predominant mechanism for other metals. The results showed that the Cr(VI) removal efficiency was 100% when nZVI was used alone without pH change, whereas it decreased to around 90% when CNTs-nZVI nanocomposite was used. On the other hand, using CNTs-nZVI showed high removal efficiency for Se and Co at 90% and 80%, respectively. The results suggest that the CNTs–nZVI nanocomposite showed high adsorption efficiency for remediation of heavy metals-contaminated water
[26]. In another study, Zhang et al.
[27] studied the performance of carboxymethyl cellulose (CMC)-stabilized nZVI composited with BC (CMC-nZVI/BC) for remediation of Cr(VI)-contaminated soil
[27]. The results indicated that, after 21 days, the immobilization efficiency of Cr(VI) was 19.7, 33.3, and 100% when the dosage of CMC-nZVI/BC was 11, 27.5, and 55 g Kg
−1, respectively. The results suggest that the addition of BC to CMC-nZVI could decrease the Cr(VI) transformation slightly, as a small part of CMC-nZVI could be adsorbed to biochar. The Cr
total removal efficiency was high because the reduction reaction continued to remediation
[28]. In a recent study, Qian et al.
[29], for the first time, investigated the performance of biochar-nZVI for the remediation of chlorinated hydrocarbon in the field
[29]. They used direct-push and water pressure-driven packer techniques. The field study results demonstrated a sharp reduction of chlorinated solvents in the 24 h after the first injection of nZVI, but within the next two weeks, a rebound of the concentrations in groundwater was observed. However, the implementation of biochar-nZVI highly improved the removal of the chlorinated solvent from groundwater for 42 days (
Figure 1). The results suggest that biochar-nZVI is a promising combined technology for chlorinated solvent contaminated groundwater remediation
[29].
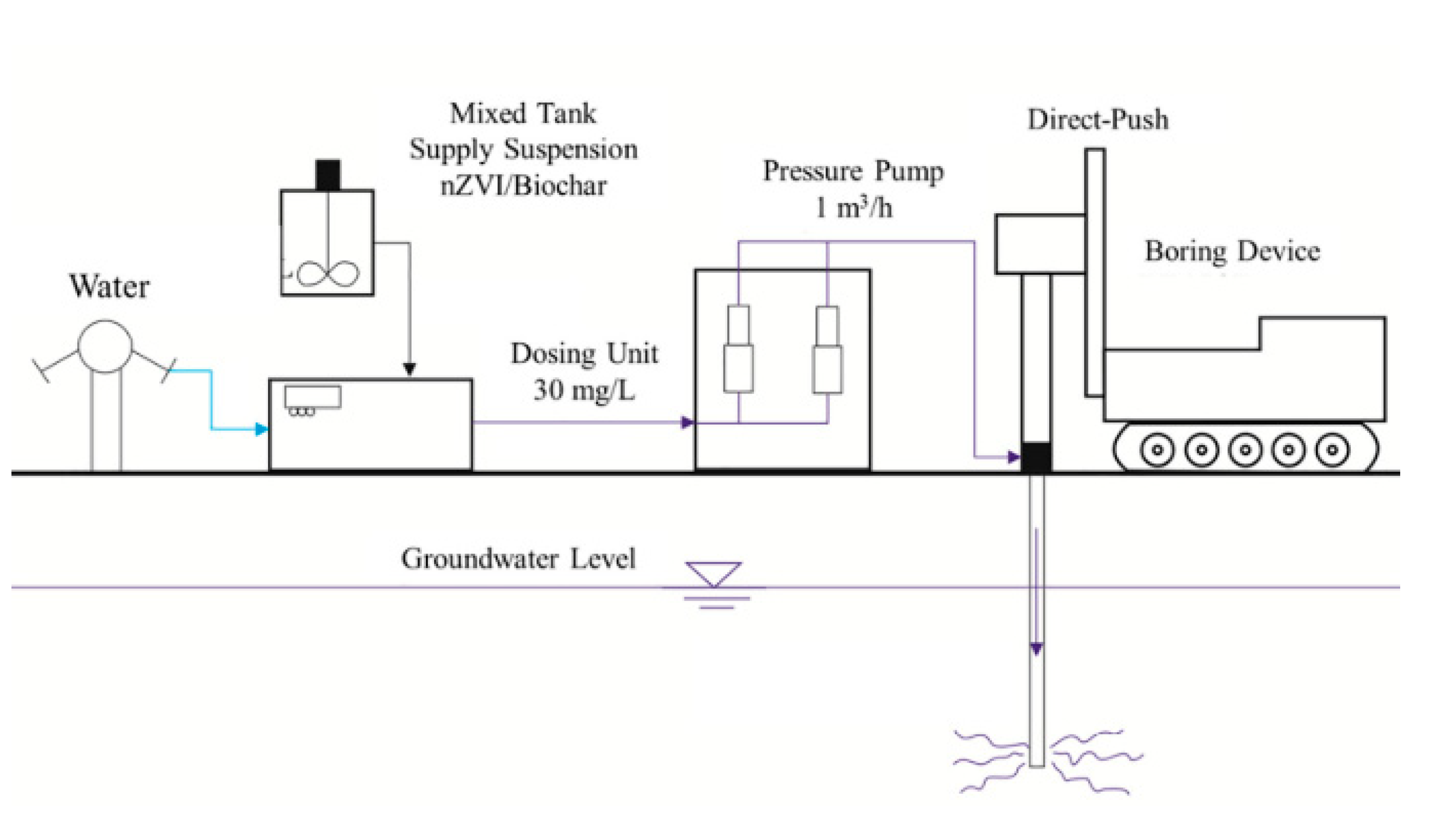
Figure 1. Step of the injection procedure. Reprinted with permission from [29] (2020, Elsevier).
Galdames et al.
[16] developed a new approach combining nanoremediation with bioremediation for hydrocarbon and heavy metals remediation from contaminated soil
[16]. Specifically, the method uses a combination of nZVI and compost from organic waste. The results indicated that the combination of nZVI and compost could decrease the aliphatic hydrocarbons concentration up to 60% even under uncontrolled conditions. In addition, they observed a remarkable decrease in ecotoxicity in the bio-pile of soil
[16]. In another study, Alabresm et al.
[30] studied the combination of PVP-coated magnetite NPs with oil-degrading bacteria for crude oil remediation at the lab scale
[30]. The result indicated that NPs alone removed around 70% of high oil concentration after 1 h. However, the removal efficiency did not increase due to the saturation of NPs. On the other hand, bioremediation by oil-degrading bacteria removed 90% of oil after 48 h. Finally, the combination of NPs and oil-degrading bacteria could completely remove the oil within 48 h. This was attributed to the sorption of oil components to NPs and following degradation by bacteria. Further investigation is needed to understand the oil removal mechanism when combining NPs with oil-degrading bacteria are used for oil remediation
[30].
Recently, Czinnerova et al.
[31] conducted a long-term field study that investigated the degradation of chlorinated ethenes (CEs) by using nZVI supported by electrokinetic (EK) treatment (nZVI-EK)
[31]. EK may enhance the nZVI impact on soil bacteria and increased the migration and longevity of nZVI. The results indicated a rapid decrease in cis-1,2-dichloroethene (cDCE) at around 70%, followed by setting new geochemical conditions as a degradation product of CE (ethene, ethane, and methane) was observed. These new conditions enhanced the growth of soil and ground bacteria, such as organohalide-respiring bacteria. The results suggest that nZVI-EK remediation technology is a promising method for CE remediation from soil and groundwater and enhanced bacteria availability in soil and groundwater
[31]. In another study, Sierra et al.
[32] studied a combination of soil washing and nZVI for the removal and recovery of toxic elements (As, Cu, Hg, Pb, Sb) from polluted soil (
Figure 2)
[32]. The results showed that a high recovery yield was obtained for Pb, Cu, and Sb in the magnetically separated fraction, whereas Hg was concentrated in a non-magnetic fraction. Taking everything into account, the soil washing efficiency was enhanced by adding nZVI, especially for a larger fraction. The results suggest that the investigated methodology opens the door for NPs’ use in soil-washing remediation
[32].
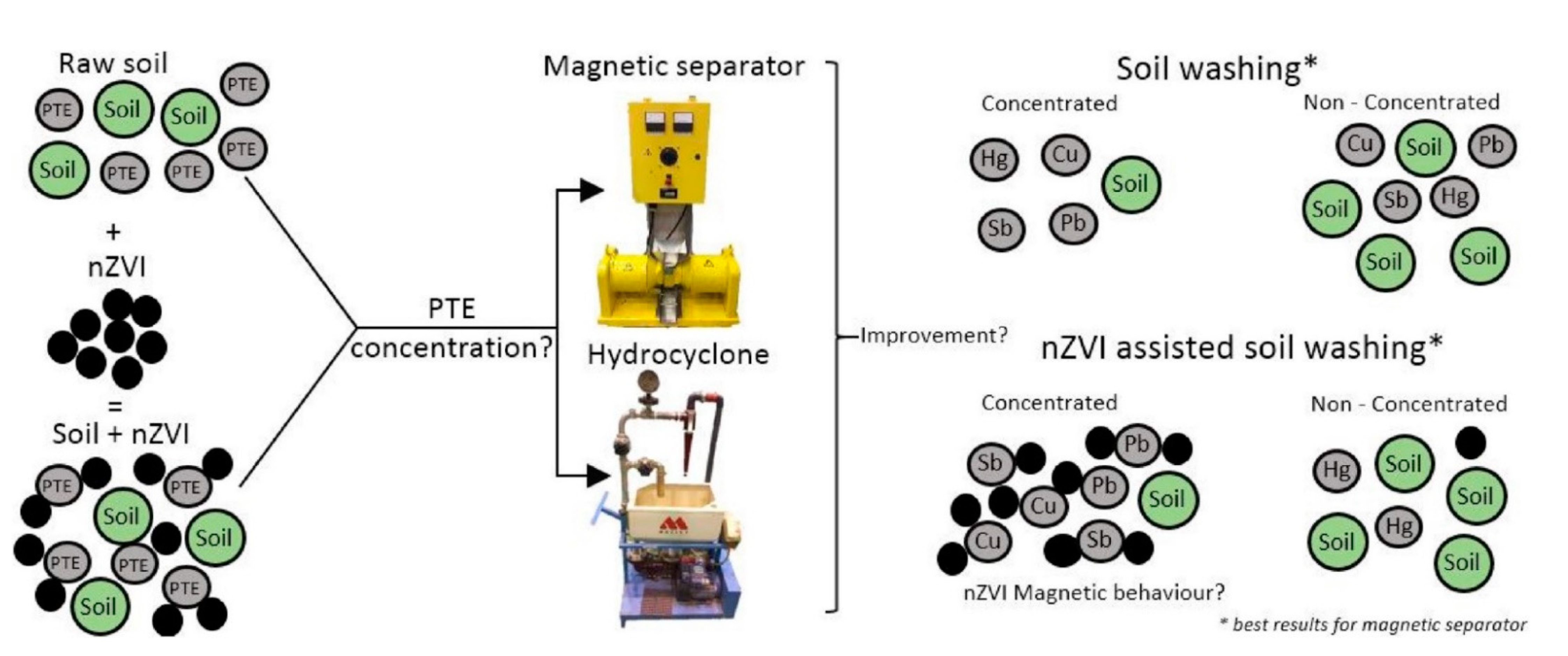
Figure 2. Soil washing assisted nZVI nanoremediation. Reprinted with permission from [32] (2018, Elsevier).
Qu et al. [33] studied the implication of an activated carbon fiber (ACF)-supported nZVI (ACF-nZVI) composite for Cr(VI) remediation from groundwater [33]. In addition, they examined the effect of the operation condition such as nZVI amount on activated carbon fiber, initial Cr(VI) concentration, and pH value on the Cr(VI) removal by conducting a batch experiment. The results indicated that the aggregation of nZVI could be inhabited by ACF, which increases the nZVI reactivity and Cr(VI) removal efficiency. The removal efficiency of Cr(VI) decreased with increasing Cr(VI) initial concentration, whereas, in an acidic environment, complete removal (100%) of Cr(VI) was observed in 1 h reaction time. The proposed removal mechanism consisted of two steps: the first step was the physical adsorption of Cr(VI) on the ACF-nZVI surface area or inner layer, where the second step was a reduction of Cr(VI) to Cr(III) by nZVI [33]. In another study, Huang et al. [34] studied the activation of persulfate (PS) by using a zeolite-supported nZVI composites (PS-Z/nZVI) system and examined its efficiency for TCE degradation. The results indicated that Z/nZVI showed high ability towards PS activation (1.5 mM), and high removal efficiency (98.8%) of TCE was observed at pH 7 within 2 h. Moreover, the PS-Z/nZVI system showed high efficiency in terms of TCE for a wide range of pH (4–7) [34].
3. Conclusions
We aim to present the latest advances in nanoremediation of contaminated soil and groundwater. The main advantages of using nanomaterials in soil remediation are reduction in cleanup time and overall costs, decreased pollutants to nearly zero in the site, and no need to dispose of polluted soil. The wide use of nZVI nanomaterials in environmental cleanup is due to their high reactivity and high ability to immobilize heavy metals such as Cd, Ni, and Pb. Modifying and/or coating nZVI may decrease the toxicity effects on soil microorganisms. The high adsorption capacity of CNTs is from the large surface area, which makes CNTs a great nanomaterial for organic and inorganic remediation. More studies are needed to investigate the effect of CNTs on the environment.
Soil and groundwater remediation using metal and MNPs is a promising technology due to the unique separation mechanism. Full-scale application of nanoremediation needs further evaluation, particularly in terms of efficiency and potential adverse environmental impacts. Combining nanoremediation with other remediation technology appears to be the future of soil remediation as the combination process increases the sustainable remediation practice towards green environmental protection practice.
This entry is adapted from the peer-reviewed paper 10.3390/w13162186