Over the years, although substantial progress has been made in understanding low-temperature response mechanisms in plants, the research is more focused on aerial parts of the plants rather than on the root or whole plant, and more efforts have been made in identifying and testing the major regulators of this pathway preferably in the model organism rather than in crop plants. For the low-temperature stress response mechanism, ICE-CBF regulatory pathway turned out to be the solely established pathway, and historically most of the low-temperature research is focused on this single pathway instead of exploring other alternative regulators.
1. Climate Change and Plant Growth
As sessile organisms, plants are unable to escape unfavorable conditions and can experience both biotic and abiotic stresses. Between these two, abiotic stress has become the most prominent cause of agricultural loss
[1,2][1][2]. Damage caused by biotic stress can be solved by manipulating single target genes or receptors. However, abiotic stresses are intertwined, which increase the plants’ susceptibility and result in more damages
[3]. For instance, the synergistic effect of drought and heat is more destructive compared to individual stress
[3,4][3][4]. Exposure to low temperatures results in mechanical constraints, alters signaling molecules, and reduces osmotic pressure at the cellular level
[5,6][5][6]. During submergence, plants experience a combination of flooding, salinity stress, and hypoxia at the same time
[7,8][7][8]. Among abiotic variables, temperature is notable because it affects almost every molecule and reaction in the cell.
Recently, temperature fluctuations have become exceptionally common across the globe. According to the latest data from the National Centers for Environmental Information, USA (NOAA), the global land surface temperature for March 2018 was 1.5 °C (2.9 °F) above average and it was the seventh highest since global records began in 1880. At the same time, cooler-than-average conditions engulfed much of Europe and western Russia during March 2018. For example, Lyon, in France, observed an average maximum temperature of 8.6 °C (47.5 °F), the lowest of March since its record began in 1938 (based on recent data of National Oceanic and Atmospheric Administration, USA). Most parts of the world are experiencing more than above-average or less than below-average temperature in the last two decades based on 140 years of temperature data (Figure 1). For instance, a comparison of 2000 and 2018 maps revealed that the earth is warming up at a dangerous pace but at the same time some areas are hit by unusual cold temperature (Figure 1). These statistics highlight the anomalous frequency of both low and high temperatures in various parts of the world in recent years.
Figure 1. Temperature anomalies around the globe. Colors indicate places where average annual temperature was above or below based on the average temperature during 1981–2010. Data source:
Climate.gov (accessed on 28 February 2022). Data provider: NOAA Environmental Visualization Laboratory (NNVL).
Cold stress is a major limiting factor for crop production worldwide, which is broadly categorized into chilling stress (0–15 °C) and freezing stress (<0 °C)
[19,20][9][10]. Additionally, to combat the cold-induced damage, some plants developed a unique process called cold acclimation where plants can acquire enhanced resistance to freezing stress when they are exposed to nonlethal low temperatures for a few days
[21][11]. Various aspects of cold stress and the underlying mechanisms linked to these processes including transcriptional regulation, calcium signaling, the role of small molecules, and epigenetic regulation have been extensively covered in some recent reviews
[22,23,24,25,26,27][12][13][14][15][16][17]. For chilling or low-temperature stress responses in plants, C-repeat-binding factors (CBF)-mediated pathway is the most studied one and is considered as the primary regulatory pathway
[28][18]. Over the last few decades, most articles related to low-temperature response echoed this idea and many of the hypotheses were validated in the model plant
Arabidopsis thaliana [28][18]. Although several articles demonstrated the existence of a CBF-independent cold response pathway
[29[19][20][21][22],
30,31,32], this pathway is much less explored, and the biological significance is still elusive.
2. Major Low Temperature-Responsive Pathway
Plants’ response to low-temperature stress starts from the plasma membrane. The immediate effect on the plasma membrane involves the alteration of fatty acid and lipid-protein interaction
[33][23]. Another major group of regulators involved in the cold perception and relaying the signal to downstream consists of calcium channels, histidine kinase, receptor kinase, and phospholipases
[31,34,35,36][21][24][25][26]. However, compared to these early cold stress perceptive regulators, a group of transcription factors has been identified to relay downstream signals and regulate a series of downstream gene expressions under cold stress. Transcription factors responding to low-temperature stress were first identified by Shinozaki’s and Thomashow’s groups in the early 1990s. This transcription factor family, which was named dehydration-responsive element binding (DREB) and C-repeat binding factor (CBF) by Shinozaki’s and Thomashow’s groups respectively, encodes DREB1A/CBF3, DREB1B/CBF1, and DREB1C/CBF2 transcription factors
[37,38][27][28].
Consistent with the prior knowledge about the DREB1/CBFs-mediated cold-induced gene expression, CRISPR-Cas9 generated single, double, and triple mutants of
DREB1/CBFs demonstrated a decrease in freezing tolerance
[39,40][29][30]. In this endeavor, two independent groups tried to find out the comparative importance among these three transcription factors for freezing tolerance. Jia et al. (2016) showed that triple mutant is extremely affected during freezing stress and ranked mutants as
cbfs >
cbf1,3 >
cbf3 for freezing sensitivity
[39][29]. In the same year, Zhao et al. (2016) also presented that triple mutant has most severe phenotype, but they ranked freezing sensitivity as
cbf123 >
cbf2 cbf3 >
cbf1 cbf3 >
cbf2 >
cbf1/cbf3 based on survival rate. The latter mentioned study emphasized the importance on DREB1C/CBF2 and suggested that DREB1C/CBF2 plays a much more important role in freezing tolerance compared to DREB1B/CBF1 and DREB1A/CBF3
[40][30]. All these results point them as master regulators of cold-inducible gene expression
[37,38,39,40][27][28][29][30].
Upstream activators/inducers of these master regulators were discovered through a series of elegant experiments. For instance,
CALMODULIN BINDING TRANSCRIPTION ACTIVATOR3/
Arabidopsis thaliana SIGNAL-RESPONSIVE GENE1 (
CAMTA3/AtSR1) acts as a positive regulator of
DREB1C/CBF2 expression, and
camta1 camta3 double mutant plants are sensitive to freezing stress
[41][31].
Another well-known regulator of
DREB1/
CBFs gene expression is
INDUCER OF CBF EXPRESSION1/SCREAM (
ICE1/SCREAM), an MYC-like basic helix-loop-helix transcription factor.
ice1, which is a dominant mutant with a single amino acid substitution at 236 (arginine to histidine R236
→H), was first isolated through a screen of a
firefly luciferase (LUC) reporter gene driven by
CBF3/DREB1A promoter. In
ice1, cold-inducible
DREB1A/
CBF3 gene expression is repressed but the expression of
DREB1B/
CBF1 or
DREB1C/
CBF2 is unaltered
[42][32].
ice1-1 mutant showed increased sensitivity to chilling and freezing response, while
ICE1 overexpressing transgenic plants,
Super-ICE1, showed an improved survival rate after the freezing treatment
[42][32]. Based on these results, an ICE1-DREB1A/CBF3 was established as a central regulatory pathway for plants’ cold stress response. Later, Kanaoka et al.
[43][33] isolated
scrm-D mutant, where the majority of the epidermal cells were transformed into guard cells. Interestingly, the mutation was found to be the same missense mutation, like
ice1-1 mutant (R236
→H)
[43][33]. They also found that SCRM1/ICE1 and SCRM2/ICE2 make heterodimers with core stomatal transcription factors. Phenotypic observation of the double mutant,
ice1-2 scrm2-1 revealed no stomatal differentiation in the epidermis
[43][33]. These findings raised some questions about the role of
ICE1 as an inducer of
DREB1A/CBF3.
3. CBF-Dependent Pathway in Crop Plant Engineering
Since the identification of CBF and CBF-dependent pathway components in the model plant
Arabidopsis thaliana, these genes are favorite targets of researchers for studying cold and chilling stress responses in plants (
Figure 2). Over the last three decades, tremendous efforts were put to generate materials, such as knockout mutants and transgenic plants expressing CBF-related genes (
Table 1). Genes from various sources were successfully transformed in Arabidopsis and other crops and variable response was observed against cold stress. Interestingly, highest number of genes for CBF regulatory pathway was found in Arabidopsis compared with other tested crop plants (
Figure 2).
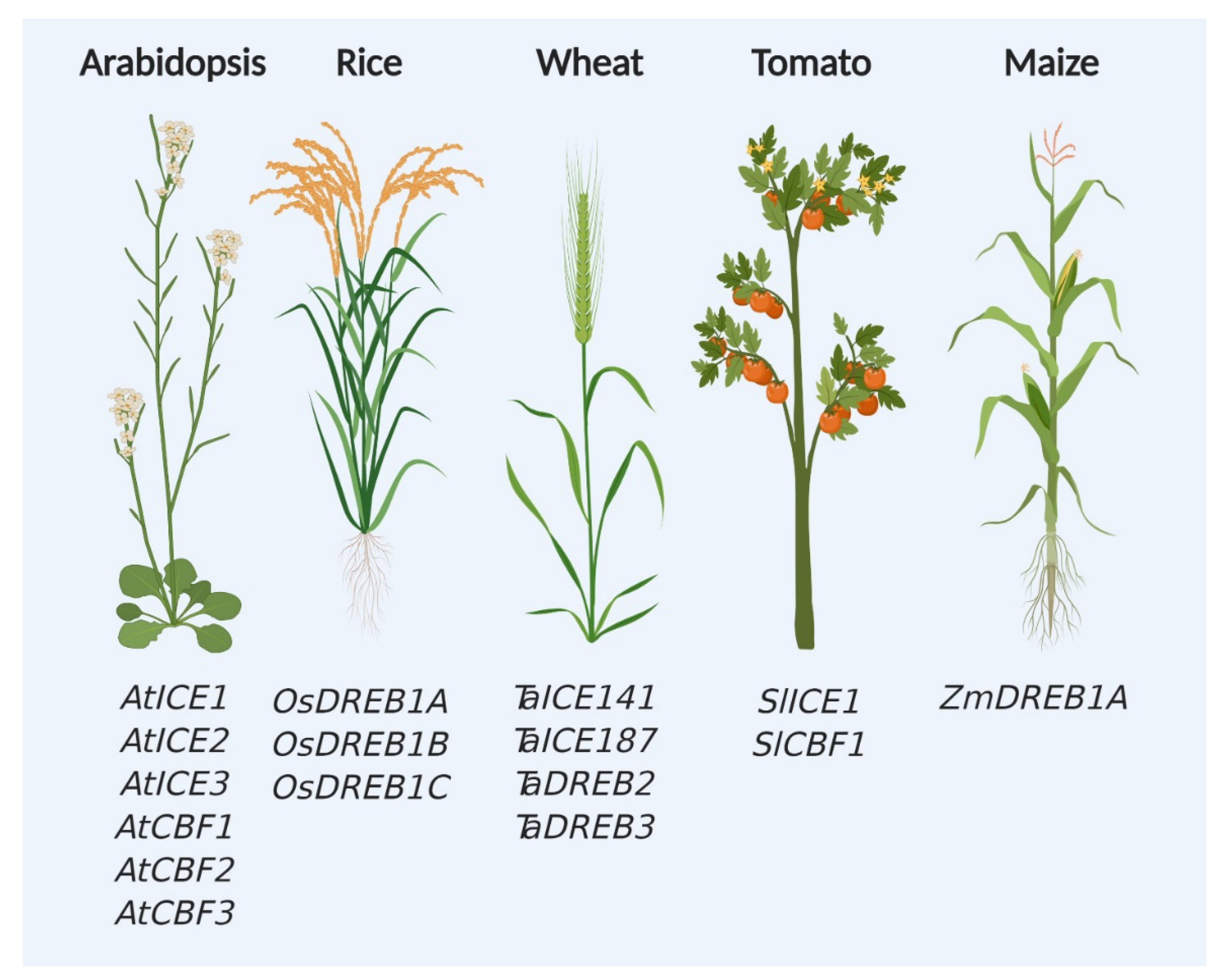
Figure 2. ICE-CBF regulators in the model and crop plants. Identified regulators from the ICE-CBF pathway are highlighted in the model (
Arabidopsis thaliana) and crop plants (
Oryza sativa, Triticum aestivum, Solanum lycopersicum, and
Zea mays).
Table 1. ICE and DREB1A/CBF identified from the model plant
Arabidopsis thaliana and crop plants are validated within the same host plant or other plants for cold tolerance or chilling stress response.
Gene
|
Source
|
Host
|
Reference
|
AtICE1
|
Arabidopsis thaliana
|
Arabidopsis thaliana
|
[42][32]
|
AtICE2
|
Arabidopsis thaliana
|
Arabidopsis thaliana
|
[45][34]
|
AtICE3
|
Arabidopsis thaliana
|
Cucumis sativus
|
[46][35]
|
AtDREB1B/
AtCBF1
|
Arabidopsis thaliana
|
Arabidopsis thaliana,
Brassica napus,
Fragaria ananassa,
Populus tremula x alba
|
[47,48,49,50][36][37][38][39]
|
AtDREB1C/
AtCBF2
|
Arabidopsis thaliana
|
Arabidopsis thaliana,
Brassica napus
|
[48,51][37][40]
|
AtDREB1A/
AtCBF3
|
Arabidopsis thaliana
|
Arabidopsis thaliana,
Brassica napus,
Solanum tuberosum,
Triticum aestivum,
Nicotiana tabacum,
Manihot esculenta
|
[48,52,53,54,55,56][37][41][42][43][44][45]
|
SlICE1
|
Solanum lycopersicum
|
Solanum lycopersicum
|
[57,58][46][47]
|
SlCBF1
|
Solanum lycopersicum
|
Arabidopsis thaliana
|
[59,60][48][49]
|
OsDREB1A
|
Oryza sativa
|
Oryza sativa
|
[61][50]
|
OsDREB1A
|
Oryza sativa
|
Arabidopsis thaliana
|
[62][51]
|
OsDREB1B
|
Oryza sativa
|
Oryza sativa
|
[61][50]
|
OsDREB1B
|
Oryza sativa
|
Nicotiana plumbaginifolia
|
[63][52]
|
OsDREB1C
|
Oryza sativa
|
Oryza sativa
|
[61][50]
|
TaICE141
|
Triticum aestivum
|
Arabidopsis thaliana
|
[64][53]
|
TaICE187
|
Triticum aestivum
|
Arabidopsis thaliana
|
[64][53]
|
TaDREB2
|
Triticum aestivum
|
Triticum aestivum
|
[65][54]
|
TaDREB3
|
Triticum aestivum
|
Triticum aestivum
|
[65][54]
|
HvCBF3
|
Hordeum vulgare
|
Arabidopsis thaliana
|
[66][55]
|
HvCBF4
|
Hordeum vulgare
|
Oryza sativa
|
[67][56]
|
ZmDREB1A
|
Zea mays
|
Arabidopsis thaliana
|
[68][57]
|
GmDREB3
|
Glycine max
|
Arabidopsis thaliana
|
[69][58]
|
VrCBF1
|
Vitis riparia
|
Arabidopsis thaliana
|
[70][59]
|
VrCBF4
|
Vitis riparia
|
Arabidopsis thaliana
|
[70][59]
|
LpCBF3
|
Lolium perenne
|
Arabidopsis thaliana
|
[71,72][60][61]
|
MbDREB1
|
Malus baccata
|
Arabidopsis thaliana
|
[73][62]
|
4. CBF-Independent Cold-Responsive Pathway
As described earlier, for the cold stress response CBF-dependent pathways always took the helm of the research. Hence, newly identified cold-responsive genes were always tested in reference to the CBF regulatory pathway. One of the major groups of genes identified for cold responsiveness is linked to the biosynthesis, transport, and signaling regulators of hormones. As most of these genes were hypothesized and experimentally looked at for their CBF-dependence, they were broadly categorized into two groups: genes functioning in CBF-dependent pathway and genes functioning in CBF-independent pathway. Interestingly, except auxin and cytokinin, all other hormonal responses under cold stress were found to be linked to CBF-dependent pathway. Since auxin and cytokinin play vital roles during plant growth and development and the genes regulating the response of these two hormones were found to be independent of the CBF regulon for cold stress-induced developmental alterations, indicating that the CBF-independent pathway may also function in parallel to CBF pathway.
One of the major CBF-independent pathways regulating cold stress response is mediated by intracellular auxin homeostasis. Earlier studies on Arabidopsis inflorescence gravity response under cold stress revealed that cold stress transiently inhibits the rootward auxin transport, which can be completely recovered after removing the cold stress
[97,98,99][63][64][65]. Cold stress-induced root growth inhibition was attributed to the altered auxin homeostasis at the root meristem, which results from the transient inhibition of the shootward auxin flow
[29][19]. Through several elegant experiments, the authors also demonstrated that cold stress inhibits the trafficking of a subset of intracellular proteins, including PIN2 and PIN3 proteins that play an indispensable role in shootward auxin transport
[29][19]. This work brought new insight into the cold stress response pathway and indicated that auxin and cellular protein trafficking may be the new players in the cold stress response pathway. This idea was substantiated by the study of Hong et al.
[32][22], where they demonstrated that reestablishment of auxin maximum is required at the quiescent center to promote the new columella stem cell daughter cells (CSDCs), which improves the roots’ ability to withstand cold stress. Consistently, it was also shown that exogenous application of IAA helps to reduce selective CSDCs death and facilitates the root growth recovery after chilling stress
[32][22].
The formation of root auxin gradient and auxin maxima solely depends on the transport of auxin, which is tightly regulated by the trafficking of a subset of PIN proteins such as PIN 1, PIN2
[100,101][66][67]. Disruption of the functional activity of these proteins either by mutation or chemical inhibitors results in altered gradient formation and maxima
[100,101][66][67]. It has already been demonstrated that these proteins continuously cycle between the plasma membrane and cytosol using several trafficking pathways. The classic experiment with a general protein trafficking inhibitor Brefeldin A (BFA) provided compelling evidence in support of PIN trafficking
[100,101][66][67]. Further, it was shown that this continuous trafficking of PINs is important for its function. Cold stress selectively inhibits the PIN2 and PIN3 trafficking resulting in altered auxin gradient, which affects the root development
[29][19]. Interestingly, this inhibition was found to be transient as the removal of cold stress restored the trafficking and the root growth recovery
[29][19]. Further exploration of the mechanism of cold-induced inhibition of protein trafficking revealed that cold stress specifically targets GNOM, a SEC7-containing ARF-GEF (Guanine nucleotide Exchange Factors for ADP Ribosylation Factor)
[30][20]. GNOM contains six characteristic domains (DCB—Dimerization and Cyclophilin Binding domain, HUS—Homology Upstream of SEC7domain, Secretory7—Catalytic domain of GEF, and HDS1–3—Homology Downstream of SEC7 domains 1–3)
[102][68] and among them, SEC7 domain is conserved across the kingdom and regulates GEF catalytic activity in the membrane
[101,103][67][69].
Partial loss of function trans-heterozygote GNOM mutant
gnomB4049/emb30−1 (
gnomB/E), where two mutations reside in the SEC7 domain
[104][70], demonstrates a hypersensitive response to cold stress. The mutations outside the SEC7 domain or mutations in GNOM LIKE (GNL) proteins show wild-type-like cold-responsive phenotype
[30][20]. In contrast, the engineered BFA-resistant transgenic GNOM line
[101][67], which contains a point mutation at 696 position (Methionine to Leucine mutation) of SEC7 domain shows strong resistance to cold stress (
Figure 3). These plants can grow and flower even when it is grown under continuous cold stress for more than a month
[30][20]. Such an elevated level of resistance of plants to cold has been demonstrated for the first time, which clearly indicates the importance of this pathway in cold stress. The biochemical and expression analyses of the BFA-resistant transgenic line revealed a surprising finding that this point mutation results in overexpression of GNOM both at transcriptional and translational levels
[30][20]. These findings leadto a hypothesis that the increased trafficking activity of GNOM may help in establishing proper auxin gradient even under cold stress, which aids the plants to withstand cold stress and grow.
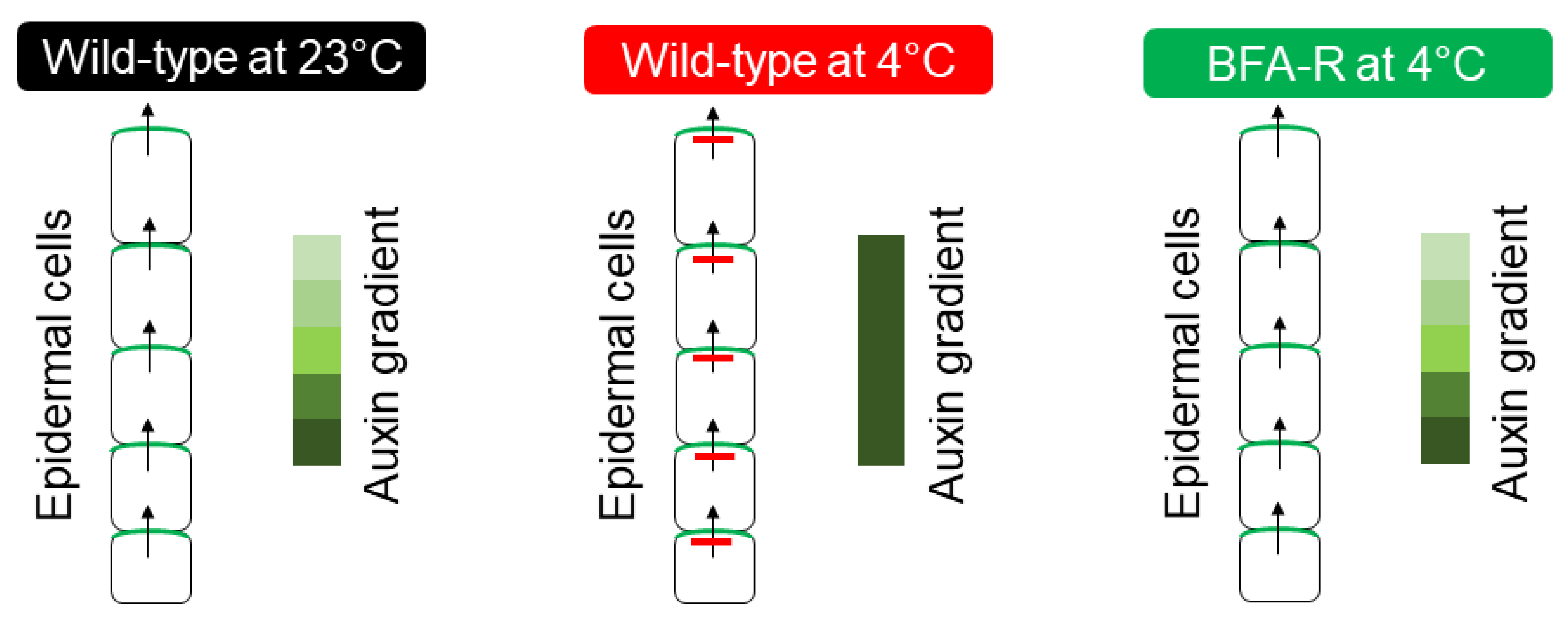
Figure 3. GNOM-mediated faster root growth recovery mechanism under cold stress. (
Left) Balanced auxin homeostasis in the epidermal cell layers of root at 23 °C. (
Middle) Low temperature-mediated inhibition of PIN2 trafficking and altered auxin gradient in the epidermal cell layers at the root. (
Right) GNOM-engineered BFA-resistant line helps to retain functional PIN2 trafficking under cold stress to maintain proper auxin gradient for root growth.
By narrowing down the cold stress response to SEC7 domain specific activity of GNOM instead of to general cellular protein trafficking, this
res
tudy earch presents a possibility that a general regulatory mechanism for cold response may exist in both plant and animal kingdom. In fact, this finding is reminiscent of evidence from yeast. In
S. cerevisiae, the P-type ATPase Drs2p is a membrane-localized protein and interacts directly and functionally with ARF GEF Gea2p. A single mutant of
drs2Δ cells is viable but has a cold-sensitive phenotype. Additionally, the double-mutant
drs2Δgea2Δ strain is even more low-temperature-sensitive. The Gea2
V698G mutant fails to interact with Drs2p and consequently becomes temperature- and brefeldin A (BFA)-sensitive
[105][71]. The study from yeast highlighted the importance of GTPase and BFA-sensitive trafficking pathways in regulating cold stress response for growth. In ARF-GEF, the catalytic domain SEC7 plays pivotal roles for GTPase activity, BFA-sensitive response, and temperature sensitivity. Yeast cells of
sec7–4, containing mutation within SEC7 domain, demonstrate reduced ARF-GEF activity and temperature sensitivity. In contrast,
sec7–1, containing mutation outside of SEC7 domain, has temperature-insensitive growth and response
[106][72] (
Figure 4). Further implicating a universal role of SEC7 domain in temperature response, SEC7 domain-containing GNOM from
Arabidopsis thaliana can rescue the temperature-sensitive yeast mutant
gea1–19gea2Δ [103][69].
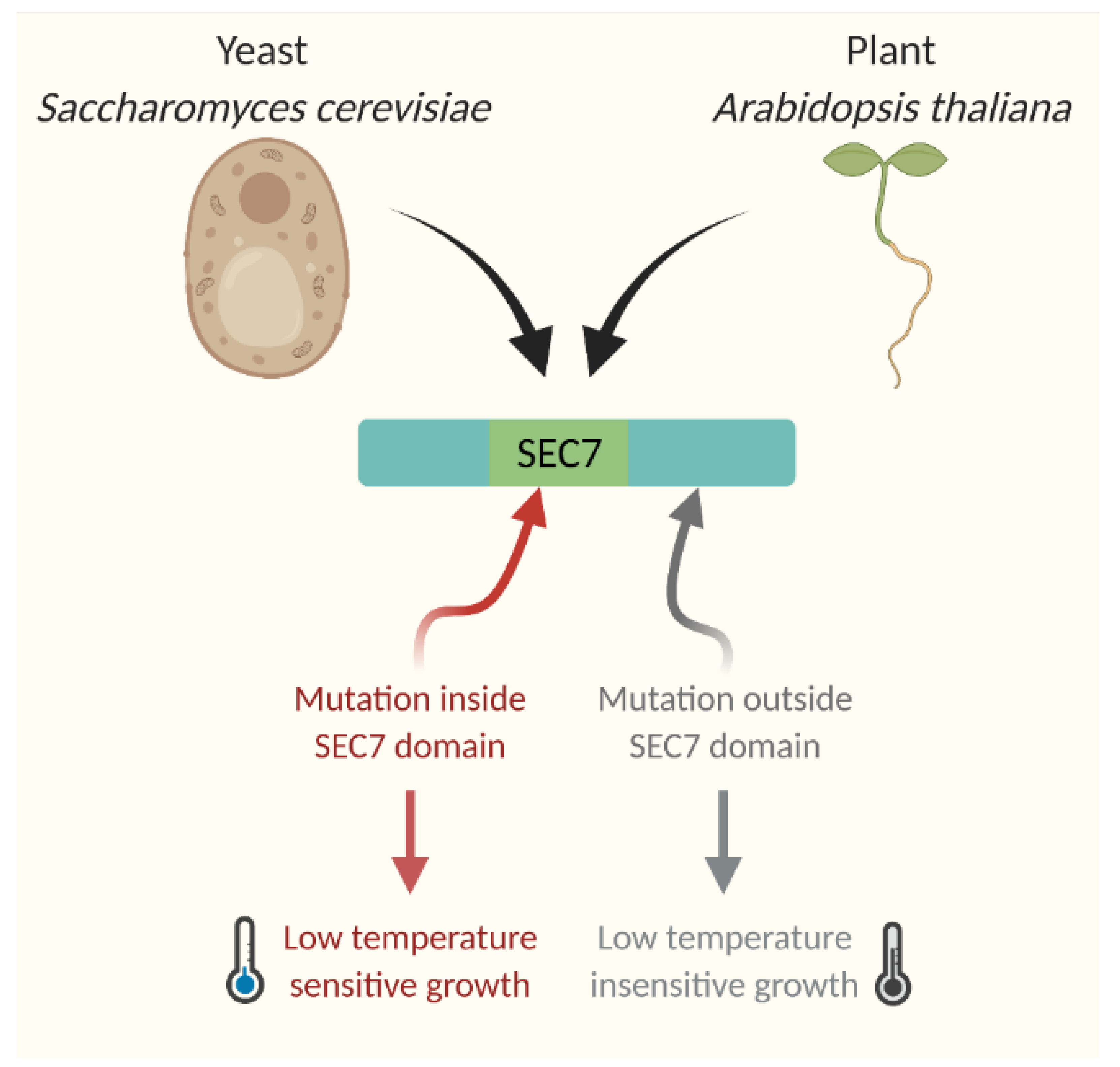
Figure 4. Role of SEC7 domain in temperature response. SEC7 domain-containing proteins are responding to low temperature in both
Saccharomyces cerevisiae and
Arabidopsis thaliana.