[1]
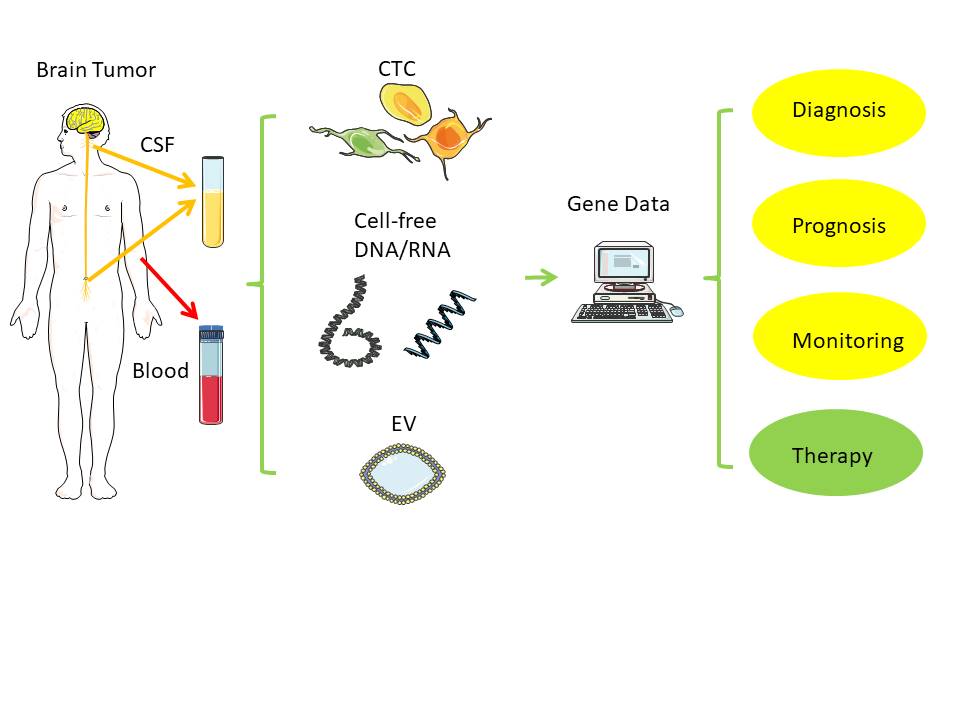
Figure 1. Liquid biopsy. Distant from the original brain tumor, samples from blood and cerebrospinal fluid (CSF) can typically serve as low-risk source of tumor-derived nucleic acids (RNA, DNA) for further analysis[1]. Notes: CSF - cerebrospinal fluid; EV - extracellular vesicle; CTC - circulating tumor cell. Created/modified with https://smart.servier.com (accessed on 8 August 2021), licensed under Creative Commons Attribution 3.0 Unported License (https://creativecommons.org/licenses/by/3.0/ (accessed on 8 August 2021)).
1. Circulating Tumor Cells—CTCs
Circulating tumor cells (CTCs) are cells derived from a tumor which enter the bloodstream and other body fluids, e.g., the CSF (
Figure 1). Unfortunately, they are extremely rare for almost all cancers; so far, a widely accepted standard method for identifying and collecting CTCs is still lacking. Since brain tumors do not usually metastasize via the bloodstream
[1][2][3][4][5][6][7], it was quite a surprise to find CTCs in 20–40% of glioblastoma patients
[7][8][9][10][11][12][13]. In carcinoma, clusters of tumor cells are increasingly associated with easier metastatic spread compared to single CTCs; similar clusters can be found in glioblastomas
[1211], supporting the idea that glioblastoma-derived CTCs can cross the blood–brain barrier as clusters of cells, although they almost never establish metastases
[1413]. Applying the seed-and-soil hypothesis from Paget from 1889, they may have the ability to spread (seed), but cannot find the right target tissue or endothelium (soil), where they can egress, survive, and grow
[1514]. The limited overall survival time of the patients may also prevent micrometastases from growing larger after seeding. Different approaches exist to isolate CTCs for many tumor entities. To isolate CTCs from carcinoma, an elegant method uses epithelial cell adhesion molecule (EpCAM) as a surface marker, which is exclusively expressed on epithelia and epithelial-derived neoplasms; since EpCAM is not expressed on leukocytes, it can be used to selectively accumulate CTCs. In contrast, EpCAM is not expressed on brain tumor cells and, therefore, cannot be used as a selection marker for these cancers. Currently, no adequate surface marker for brain tumor CTCs has been established. Unfortunately, other isolation methods, which use the bigger size of tumor cells, are still less efficient. However, in at least 50% of the cases, CTCs from brain tumors can in fact be isolated from the blood or CSF. These can be extremely informative for molecular diagnostics with subclassification of tumors, as well as prognosis and repeated monitoring of progression and therapy resistance. The specificity of mutations found in CTCs is very high, but low sensitivity remains a big challenge, i.e., not all liquid biopsies from brain tumor samples allow the isolation of CTCs. Not only the genetic analysis of CTCs is tempting to be applied for diagnostics, but also pharmacological and
[1615] tests are in progress
[16][17][18][19][20][21][22][23][24][25][26]. It is noteworthy that brain tumor CTCs are easier to collect from CSF than from blood. Interestingly, cisternal puncture appears to give even better results than lumbar puncture, although the CSF is moving downward and is fully exchanged 3–5 times per day. Interestingly, circulating epithelial, i.e., noncancerous, cells have also been found in benign, inflammatory bowel diseases
[2726]; this points to the need for molecular characterization of cells obtained for CTC testing. Collection of CSF is usually performed easily, with a rare risk of damage to the brain. Major challenges for CTCs in brain tumors are the low sensitivity and the need for standardization, e.g., the amount of material and the source (blood, serum, plasma, or CSF from lumbar or cisternal puncture). If possible, cisternal puncture appears to be superior, but this procedure is not as easily performed as a lumbar puncture. For blood samples, 7.5 mL may become a standard for some, although higher amounts of blood increase the chance of finding CTCs, which is why others recommend over 30 mL. Due to these limitations, CTCs are only useful for a few patients with brain tumors. The collection of a sufficient amount of CSF may appear to be an additional challenge for children with brain tumors, such as medulloblastomas, but usually not for adults; it is also possible to use a previously implanted shunt from the ventricle to collect CSF. In carcinomas, the number of CTCs was correlated with time of survival, i.e., patients with more than a certain number died early, while others survived up to 10 times longer
[2827].
2. Cell-Free DNA and Circulating Tumor DNA
In 1948, two French scientists found nucleic acids in human blood
[2928], but only 60 years later was this finding applied to detect specific mutations found in colon cancer
[3029]. In cancer patients, circulating tumor DNA (ctDNA) represents only a fraction of the total cell-free DNA (cfDNA) (
Figure 1). In a xenograft model, the principal fragment length of human glioblastoma ctDNA was typically shorter than the background rat cfDNA, 134–144 bp vs. 167 bp, respectively
[3130]. Such distinct differences between normal cfDNA and tumor-derived ctDNA allow selection for the shorter cfDNA to increase sensitivity.
Earlier studies showed that specific mutations well known from tissue biopsies
[31][32][33][34][35] can also be detected in serum
[35][36][37][38][39], in plasma
[39][40][41][42], or in both
[3736]. More recent studies changed from searching for single, specific mutations to sequencing panels of tumor-related genes in plasma
[42][43][44][45]. CSF showed a significantly higher sensitivity than serum or plasma in such multigene assays
[45][46][47][48][49][50]. With next-generation sequencing (NGS), it was shown that CSF-derived ctDNA represented genomic alterations of brain tumors better than blood-derived ctDNA
[5150]. The first detection of frequent and important histone H3 mutations in CSF in children with usually unresectable midline glioma supports the clinical utility of such an approach
[5251], since CSF is more safely accessible than tissue biopsy from the brainstem or thalamus.
Despite a high specificity to detect tumor-associated mutations in ctDNA from blood or CSF, variable sensitivity limits the use of ctDNA for routine clinical applications
[5049][5251]. Independent of tumor size, entity, and grading, a close location to a neighboring CSF reservoir correlated with a higher sensitivity to detect the ctDNA of medulloblastomas, ependymomas, and high-grade gliomas
[5049], although, surprisingly, not all tumors (ependymoma, low-grade glioma) abutting CSF space were detectable in this way. However, under certain conditions, liquid biopsy can be beneficial for some patients in order to (1) differentiate between pseudoprogression and real tumor progression, (2) monitor tumor response after surgery, chemotherapy, or radiation therapy, or (3) monitor tumor relapse before image diagnostics.
Over two decades, several mutations and detection methods from blood and CSF have evolved (more or less) chronologically (
Table 1). The initial focus was on methylation-specific PCR of the MGMT promoter, p16, DAPK, RASSF1A, p73, PTEN promoter, p15INK4B, and p14ARF, as well as the LOH of 10q, 1p, and 19q, and sequencing specific mutations of PTEN, IDH1/2, EGFR, TP53, PIK3CA, EPHB1, telomerase reverse transcriptase (TERT), ANK, FTH1, OR51D1, NF2, AKT1, Met, ATRX, H3F3H, HIST1H3B, BRAF, JAK2, NF1, NRAS, GNAS, ATM, 1P19Q, and CIC
[35][36][37][38][39][40][41][4248][49][5052][53][54][55]. More recent studies analyzed a number of genes by sequencing panels of genes from 54–70 genes up to whole-genome analysis
[4140][4443][4847]. Some of these typical mutations known from surgical biopsies are also relevant for therapeutic decisions
[55][56][57]. Detection limits vary, and sensitivity appears to be better from CSF. Currently, a standard to use ctDNA in brain tumors needs to be established.
Table 1. ctDNA markers tested in liquid biopsy of brain tumors.
Year |
Gene |
Variation |
Source |
Method |
Tumor |
2003 [3635] |
MGMT (promoter) |
Methylation |
Serum |
MS-PCR |
GBM |
2006 [4241] |
Methylation |
Plasma |
MS-PCR |
GBM, AA |
2010 [3837] |
Methylation |
Serum |
MS-PCR |
Astrocytic tumors (WHO III, IV), oligodendroglial tumors (WHO II, III) |
2013 [3938] |
Methylation |
Serum |
MS-PCR |
Glial tumors (II, III, IV), meningioma |
2003 [3635] |
p16 |
Methylation |
Serum |
NGS |
LGG, GBM |
3. MicroRNA—miRNA—miR
MicroRNAs (miRNA, miR) are only 20–24 nucleotides long, i.e., very small, noncoding RNA molecules derived from just 1% of the whole genome. They are strongly involved in regulation of the stability and translation of mRNA in health and disease. Although first found in 1993 in the nematode
Caenorhabditis elegans [6059], the potential biological effects of up to 1900 miRNAs in humans are not completely understood. Many seem to play a role in tumor biology, angiogenesis and immunology and some can be considered as promising prognostic factors or as potential therapeutic targets in glioblastoma (
Table 2)
[5352].
Most of the over 20 studies on miRNAs in gliomas showed variable, reasonable degrees of sensitivity and specificity, both often over 80% to 90%. The miRNAs relevant for brain tumors are often upregulated with a worse prognosis, but can also be downregulated compared to others: miR-10b, miR-15b, miR-15b-5p, miR-16-5p, miR-19b-3p, miR-20a-5b, miR-20a-5p, miR-21, miR-23a, miR-29, miR-106a-5p, miR-125, miR-128, miR-125, miR-125b, miR-128, miR-130-3p, miR-133a, miR-145-5p, miR-150, miR-181b-5p, miR-182, miR-182-5p, miR-197, miR-205, miR-208a-3p, miR-210, miR-221, miR-222, miR-222-3p, miR-223, miR-320, miR-320e, miR-328-3p, miR-339-5p, miR-340-5p, miR-374-3p, miR-376a, miR-376b, miR-376c, miR-454, miR-485-3p, miR-486, miR-486-5p, miR-497, miR-543, miR-548b-5b, and RNU6-1
[60][61][62][63][64][65][66][67][68][69][70][71][72][73][74][75][76][77][78][79][80]. Upregulation of miR-21 may serve as an early diagnostic but also as prognostic
[6362] and monitoring marker
[8180], whereas panels of different miRNAs were found to be potential markers for diagnostics and tumor grade, as well as prognostics
[8281]. In an elegant new model, urine samples from mainly glioma patients and noncancer individuals were used to develop with artificial intelligence (AI) a diagnostic model for the detection of such tumors in urine samples
[8382]. A panel of 23 miRNAs was found to separate noncancerous from glioma patients. However, a common standard needs to be established and validated to diagnose tumor patients not only from noncancer individuals, but also from patients with other diseases, such as inflammations or degenerating diseases. Future studies may also include circular RNAs (circRNA) as possible markers. They are more stable than single-stranded RNA, and some of them can serve as a functionally antagonistic sponge for specific miRs and, therefore, are significantly involved in gene regulation
[5453].
Table 2. Examples of circulating miRNA markers in brain tumors.
Year |
miR |
Variation |
Source |
Method |
Tumor |
2016 [8180] |
miR-10-b |
Up/progression |
Serum |
qPCR |
HGG |
miR-21 |
2016 [7069] |
miR-205 |
Down/diagnostics |
Serum |
qPCR |
Glioma |
2018 [6766] |
Panel of 7 miRNAs |
Diagnostic signature |
Serum EV |
NGS |
GBM |
MS-PCR |
2020 | GBM |
[6564] |
miR-21 |
Up/progression |
Serum |
ddPCR |
LGG, GBM |
2006 [4241] |
Methylation |
Plasma |
MS-PCR |
GBM, AA, AOA |
2003 [ |
miR-20e3635] |
DAPK |
Methylation |
Serum |
MS-PCR |
GBM |
miR-223 |
2003 [3635] |
RASSF1A |
Methylation |
Serum |
MS-PCR |
GBM |
2020 [6665] |
miR-17-5p |
Up/progression |
Serum |
qPCR |
GBM |
2013 [3938] |
Methylation |
Serum |
MS-PCR |
Glial tumors (II, III, IV), meningioma |
miR-125b |
2006 [4241] |
p73 |
Methylation |
Plasma |
MS-PCR |
GBM |
miR-221 |
2010 [3837] |
PTEN |
2020 | Methylation |
[8483 | MS-PCR |
]MS-PCR |
Astrocytic tumors (WHO III, IV) |
miR-486 |
Up/diagnostic |
EV from tumor microenvironment/neurosurgical aspirate fluid |
NGS |
GBM |
2014 [3736] |
Mutation |
Plasma, serum |
Digital PCR, sequencing |
Glioma II, AA, GBM |
2021 [8584] |
miR-21 |
Up/progression |
Serum EV |
qPCR |
HGG |
2010 [3837] |
10q |
LOH |
Serum |
LOH |
Astrocytic (WHO III, IV), Oligodendroglial (WHO II, III) |
miR124-3p |
2010 [3837] |
1p |
LOH |
Serum |
LOH |
Oligodendroglial (WHO II, III) |
miR-222 |
2010 [3837] |
19q |
LOH |
Serum |
LOH |
Oligodendroglial (WHO II, III) |
2021 [8382] |
Panel of 23 miRNAs |
Screening signature |
Urine |
nanowire |
GBM, glioma |
2012 [4039] |
IDH1 |
Mutation (R132H) |
Plasma |
digital PCR |
Glioma (WHO grade II, III, IV) |
2014 [3736] |
Mutation |
Plasma, serum |
Digital PCR, sequencing |
Glioma II, AA, GBM |
2013 [3938] |
p15INK4B |
Methylation |
Serum |
MS-PCR |
Glial tumors (II, III, IV), meningioma |
2013 [3938] |
p14ARF |
Methylation |
Serum |
MS-PCR |
Glial tumors (II, III, IV), meningioma |
2014 [3736] |
TP53 |
Mutation |
Plasma, serum |
Digital PCR, sequencing |
Glioma II, AA, GBM |
2014 [3736] |
EGFR |
Mutations |
Plasma, serum |
Digital PCR, sequencing |
Glioma II, AA, GBM |
2014 [3736] |
PIK3CA |
Mutation |
Plasma, serum |
Digital PCR, sequencing |
Glioma II, AA, GBM |
2015 [5150] |
TP53 (R114C) EPHB1 TERT PIK3CG IDH1 (R132H) ANK (K2337X) EGFR (C620S) PTEN (D162) FTH1 (R108K) OR51D1 (R135C) |
Mutations |
CSF, (plasma) |
ddPCR, MAF |
GBM |
2015 [5049] |
Genome |
Mutations |
CSF |
TAS/WES |
AA III, PA I, ependymoma, medulloblastoma IV, GBM, LGG II, diffuse astrocytoma |
2015 [4948] |
Gene panel (587 genes) including NF2, AKT1 |
Mutations |
CSF, plasma, serum |
ddPCR/TAS |
Vestibular schwannoma, atypical meningioma |
2017 [4140] |
Gene panels (54, 68, 70 genes) including p53, EGFR, Met |
Mutations |
Plasma |
NGS |
Brain tumors (not specified) |
2018 [4645] |
IDH1, IDH2, TP53, TERT, ATRX, H3F3A, HIST1H3B |
Mutations |
CSF |
sequencing |
Diffuse gliomas |
2018 [4847] |
Genome |
SCNAs and fragmentation |
CSF |
WGS |
Glioma |
2018 [5857] |
TERT |
Mutation |
CSF, (plasma) |
PCR, sequencing |
GBM |
2019 [5958] |
BRAF |
Mutation (V600E) |
CSF, plasma, serum |
dPCR |
PXA, ganglioglioma, PA, pilomyxoid astrocytoma |
2019 [4443] |
Genome including TP53, JAK2, NF1, EGFR, BRAF, IDH1, NRAS, GNAS, ATM |
Mutations |
Plasma |
NGS |
Astrocytic/oligodendral tumors grades I–IV, including GBM, medulloblastoma, meningioma, and ependymoma |
2019 [4746] |
IDH1 1P19Q CIC ATRX TP53 |
Mutations |
CSF |
4. Extracellular Vesicles—EVs
Tumor and normal cells can release small, extracellular vesicles into body fluids, such as blood and CSF (
Figure 1). In addition to proteins, these vesicles contain DNA and RNA, including miRNA, which are protected by the cellular membrane. EVs can be analyzed to reliably detect tumor-specific mutations, including amplification of wild-type EGFR
[85][86][87][88]. CSF appears to have an advantage over serum, perhaps due to the reduced number of EVs from leukocytes compared to blood. For example, IDH1 mutation G395A was detected in CSF-derived EVs of glioma grades II, III, and IV with a sensitivity of 63% and a specificity of 100%, but not in frozen serum
[5554]. Using quantitative PCR changes in wildtype IDH1 levels can also be used to monitor tumor burden and treatment response when the tumor does not have an IDH-1 mutation
[5554]. Most glioblastomas have an amplification of the wild-type epidermal growth factor receptor gene (EGFR), which results in an increased RNA expression; this amplification can be detected (indirectly) by quantitative reverse transcriptase-PCR (qRT) of CSF-derived EV RNA
[8685]. Using the same method, another typical mutation in glioblastomas can be detected, EGFRvIII, which lacks several exons. This deletion mutant was also detected in EV from blood in high-grade gliomas (III and IV)
[86][87][88] and may serve as a good biomarker. EVs from serum or from neurosurgical fluid were also used to detect miRNAs from glioblastomas
[6766][8483].