1. Introduction
Nucleated human cells are separated from the environment by the so-called plasma membrane and contain different subcellular compartments, called cell organelles (
Figure 1). These organelles are surrounded and, thereby, separated from the aqueous, albeit gel-like, cytosol by at least one biological membrane and have to be distributed to daughter cells from the mother cell during cell division (with the exception of lipid droplets and peroxisomes). In the cytosol, the vast majority of the approximately 24,000 different polypeptides of human cells are synthesized by 80S ribosomes. Therefore, the distinct proteins of the various organelles and the plasma membrane have to, first, be targeted to the specific organelles and, subsequently, inserted into or translocated across the membrane(s) of the relevant organelles. The protein import into the organelle termed the endoplasmic reticulum (ER) is the first step in the biogenesis of about one-third of the different soluble and membrane proteins (MPs) of human cells and, therefore, represents a central cell biological research topic of the past fifty years as well as several years to come. In a second step, the non-ER proteins reach their functional location in either the extracellular space; one of the endocytotic or exocytotic organelles (ERGIC, Golgi apparatus, endosome, lysosome, or trafficking vesicles); the plasma, peroxisomal, and mitochondrial membrane or in lipid droplets by vesicular transport; direct budding of new organelles (peroxisomal precursors or lipid droplets); or the ER–SURF pathway (mitochondria)
[1][2][3][4][5][6][7][8][9][10]. The first insights into ER protein import were gained about seventy years ago. From electron microscopic images, Palade and Potter concluded that the ER represents a ‘continuous, tridimensional reticulum’ and that ‘the surface appears to be dotted with small, dense granules that cover them in part or in entirety’, i.e., cytosolic 80S ribosomes
[11][12].
Figure 1. 3D reconstructions of a typical nucleated human cell and a ribosome-bound Sec61 translocon. The figure and its legend were adapted from Lang et al. and Sicking et al.
[5][6]. The upper part shows an artist’s view of a 3D reconstruction after live cell fluorescence imaging with ER-resident GFP and mitochondrial RFP and the central part a 3D reconstruction of the native ribosome-translocon complex in the human ER membrane after cryoelectron tomography. In human cells, the heterotrimeric Sec61 complex together with the ribosome form various large multicomponent complexes, e.g., the most abundant one comprising the multimeric membrane proteins translocon-associated protein (TRAP) and oligosaccharyltransferase (OSTA), which catalyzes N-linked glycosylation. This super-complex, now termed OSTA translocon, can insert into the membrane or translocate into the lumen a whole variety of topologically very different precursor polypeptides, i.e., type I-, type II-, glycosylphosphatidylinositol-, or GPI-anchored and type I multispanning membrane proteins, as well as soluble proteins, respectively (
Figure 2). Membrane insertion and translocation are facilitated by either a cleavable amino-terminal SP or the TMH of the nascent precursor polypeptide, which acts as a non-cleavable SP substitute (
Figure 2). The lowest part represents a modeling of reversible gating of the heterotrimeric Sec61 channel by SPs or TMHs. The fully open state of the Sec61 channel allows the translocation of hydrophilic domains of MPs or entire precursor polypeptides from the cytosol into the ER lumen (via the aqueous channel pore) and the insertion of transmembrane domains into the ER membrane (via the lateral gate), respectively.
The latter observation paved the way for the ‘signal hypothesis’ by G. Blobel and colleagues
[1][2][13][14], which suggested that topogenic sequences in nascent precursor polypeptides guide the translating ribosomes to the ER membrane and that the subsequent membrane insertion or translocation occurs coupled to translation, i.e., cotranslationally (
Figure 1). For membrane proteins, the beauty of this concept of cotranslational ER protein import is that their eventual hydrophobic transmembrane domain or domains do not face the problem of aggregation in the cytosol. Subsequent work in human cell-free systems and in the yeast
Saccharomyces cerevisiae uncovered that the topogenic sequence, termed the amino-terminal signal peptide (SP) or SP-equivalent transmembrane helix (TMH), of a nascent precursor polypeptide is recognized and bound by the cytosolic signal recognition particle (SRP), which facilitates the association of the complex between the ribosome, nascent chain, and SRP with the heterodimeric SRP receptor in the ER membrane, termed the SR (
Figure 2 and
Figure 3)
[2][15][16][17][18][19][20][21][22][23][24][25][26][27][28]. Thus, the combined action of SRP plus SR represents an ER targeting pathway for nascent precursors of soluble and membrane proteins, as well as the corresponding mRNAs. Recently, proximity-based ribosome-profiling experiments confirmed the preference of SRP and SR for SPs and relatively amino-terminal TMHs of the nascent precursor polypeptide chains
[29][30]. Typically, the interaction of SRP with SR leads to the cotranslational transfer of the ribosome-nascent chain complex (RNC) to the central component for both protein translocation and membrane insertion in the ER membrane, the translocon, or the heterotrimeric Sec61 complex (
Figure 1)
[31][32][33][34][35][36][37][38][39][40][41]. SPs and TMHs of nascent precursors may spontaneously interact with and trigger the opening of the Sec61 channel; i.e., both the central aqueous channel, as well as the lateral gate (
Figure 1), or the productive Sec61 interaction may be facilitated by one of the auxiliary components of the ER membrane, i.e., the heterotetrameric translocon-associated protein (TRAP)
[42][43][44][45][46][47][48], the heterodimeric Sec62/Sec63 complex with or without the help of the ER lumenal chaperone BiP
[49][50][51][52][53][54][55][56][57][58][59][60][61][62], and the translocating chain-associated membrane protein 1 (TRAM1) (
Figure 1 and
Figure 3)
[63][64][65][66][67]. Notably, however, there is a human paralog of the α-subunit of the Sec61 complex, termed Sec61α2, i.e., a putative alternative Sec61 complex that is more or less uncharacterized but recently addressed with respect to its substrates or client spectrum in yeast
[68], and there exist alternative components for the targeting (SND, TRC/GET, and PEX3)
[69][70][71][72][73][74][75][76][77][78][79][80][81][82][83][84][85][86][87][88][89][90][91][92][93][94][95][96][97][98][99][100][101][102][103][104][105][106][107][108][109][110][111][112][113][114][115][116][117][118][119][120], as well as membrane insertion of precursors (EMC and the GEL-BOS-PAT complex)
[121][122][123][124][125][126][127][128][129][130][131][132].
Figure 2. Topogenic sequences in precursors of soluble and membrane proteins for import into the human endoplasmic reticulum (ER). The cartoons depict signal peptides (SPs, shown in red) and seven classes of ER membrane proteins (MPs), plus their membrane protein types (in bold face) and potential topogenic sequences. Cleavable SPs have a tripartite structure (including a hydrophobic or H-region with 7–9 amino acid residues, or aas for short) and facilitate ER import of soluble proteins, GPI-anchored MPs, and single-spanning type I MPs (all shown in green). In addition, they mediate ER import of some multispanning MPs, but not of hairpin, single-spanning type II or III, TA, and other multispanning MPs (which can also be of type II or III; by definition, the shown multispanning MP is of type III). All the latter MPs depend on more or less amino-terminal transmembrane helices (TMHs, with a length of 15–25 amino acid residues) that serve as SP equivalents and facilitate membrane targeting, as well as membrane insertion. Notably, precursors of soluble proteins with SP and of type II or III and TA MPs contain only one topogenic sequence, whereas, in all other precursors, other TMDs than the TMHs may serve as alternative or additional topogenic sequences. Positively charged amino acid residues (+) play an important role in the orientation of SPs and MPs in the ER membrane, where the orientation follows the positive inside rule. Cleavable SPs are removed from the precursor polypeptides in transit by one of the two signal peptidase complexes (SPCs), which have their catalytic sites in the ER lumen. Following ER import and simultaneous cleavage of the SP, as well as the C-terminal GPI-attachment sequence, GPI-MPs become membrane-anchored via carboxy-terminal GPI attachment. Notably, HP proteins are also termed monotopic MPs, and type II and type III MPs are also referred to as MPs with N
in or N
cyt and N
out and N
exo, respectively, signal anchors
[1][69][70]. The figure and its legend were adapted from Lang et al. and Sicking et al.
[5][6]. C, carboxy-terminus; GPI, glycosylphosphatidylinositol; N, amino-terminus.
Figure 3. Components that are involved in protein import into the endoplasmic reticulum (ER) of human cells. ER protein import comprises a targeting step, plus a translocation or membrane insertion step, and may involve targeting of receptors for mRNAs or ribosomes with or without short nascent polypeptide chains to the ER (LRRC59, RRBP1, AEG-1, or KTN1). Alternatively, nascent or fully synthesized precursor polypeptides are targeted to the ER, depending on their topogenic sequences and targeting pathways, which involve cytosolic components, as well as their corresponding heterodimeric receptors in the ER membrane, such as SRα/SRβ, Snd2/Snd3, PEX3/PEX16, and Wrb/Caml. The membrane translocation is mediated by the heterotrimeric and polypeptide-conducting Sec61 channel, which may be supported by either the TRAP complex or the Sec62/Sec63 complex. Membrane insertion is mediated by several membrane protein insertases, i.e., (i) the Sec61 channel; (ii) the Sec61 channel with its partner complexes GEL, BOS, and PAT; (iii) the multimeric EMC; or (iv) the heterodimeric Wrb/Caml complex. The long arrow (in magenta) points to the open aqueous channel and open lateral gate, respectively, of the fully open Sec61 channel; the short arrows (in magenta) point to the characteristic hydrophilic vestibules of the MP insertases. Notably, (i) according to structural prediction tools, the yeast and human Snd2 may also be able to form a hydrophilic vestibule near the cytosolic surface of the ER membrane and, therefore, may also be able to facilitate membrane insertion
[74][106]; (ii) the TIGER domain represents a cytosolic micro-domain, enriched in MP-encoding mRNAs with multiple AU-rich elements or AREs in their 3′UTRs in the vicinity of the ER
[109][110]; and (iii) PEX3 is present in an ER subdomain which may be identical to the pre-peroxisomal ER
[71][72][73]. The figure was adapted from Tirincsi et al.
[106].
2. Proteins of the ER Membrane
The SPs, mentioned above, have a tripartite structure (including an amino-terminal or N-region, a hydrophobic or H-region, and a carboxy-terminal or C-region) and facilitate the ER targeting of soluble proteins, GPI-anchored MPs, and single- as well as multispanning type I MPs (
Figure 2)
[15][16][17][69][70]. In contrast, all the other MPs of the ER membrane (hairpin or HP proteins, and single- and multispanning type II or type III MPs, as well as TA proteins) depend on more or less amino-terminal or even carboxy-terminal TMHs (with a typical length of 15–25 amino acid residues) that serve as SP equivalents and facilitate membrane targeting as well as membrane insertion
[15][16][17]. HP- and GPI-anchored proteins are special
[71][72][73][74][75]: Following ER import and the simultaneous cleavage of the SP, as well as the carboxy-terminal GPI-attachment sequence, GPI-anchored MPs become membrane-anchored via carboxy-terminal GPI attachment. HP proteins do not contain a real transmembrane domain (TMD); instead, they comprise one or more hydrophobic stretches of amino acid residues, which are typically defined by databases as TMDs and, therefore, discussed here as such. By definition, precursors of soluble proteins with SP and of single-spanning type II or III and TA MPs contain only one topogenic sequence, whereas, in all other precursors, other TMDs (i.e., other than the most amino-terminal TMHs) may serve as alternative or additional topogenic sequences. Typically, SPs are removed from the precursor polypeptides in transit by signal peptidases with ER lumenal catalytic sites
[76][77][78]. Furthermore, many of the polypeptides of the secretory pathway become N-glycosylated by one of the two oligosaccharyltransferases (OST), which also have their catalytic sites in the ER lumen but act either on precursors in transit (i.e., cotranslationally, OSTA,
Figure 1) or posttranslationally (OSTB)
[79][80][81].
3. Targeting of Precursor Polypeptides to the ER Membrane
The characterization of precursors capable of SRP-independent ER targeting, such as small presecretory proteins and TA proteins, first suggested the existence of SRP-independent ER targeting pathways to the ER
[82][83][84][85][86][87]. In contrast to the SRP/SR pathway, these alternative targeting pathways can direct precursor polypeptides to the Sec61 complex co- as well as posttranslationally, and are named the TRC or GET, PEX19/PEX3, and Snd2/Snd3 pathway (
Figure 3)
[58][71][73][74][75][87][88][89][90][91][92][93][94][95][96][97][98][99][100][101][102][103][104][105][106]. Notably, there is also SRP-independent targeting of all sorts of mRNAs to the ER surface (
Figure 3)
[29][107][108][109][110]. Typically, this mRNA targeting to the ER depends on receptors for mRNAs (such as KTN1), or RNCs with nascent polypeptide chains that are not yet long enough to allow the interaction of the topogenic sequence with SRP (such as RRBP1, LRRC59, and AEG-1) (
Figure 3)
[29][111][112][113]. In the case of the ER targeting of mRNAs that code for cytosolic proteins, the nascent polypeptide-associated complex (NAC) can bind to the amino-terminus of the nascent polypeptides and trigger their release from the Sec61 complex
[23][114][115][116].
4. Insertion of Precursor Polypeptides into the ER Membrane
As stated above, the insertion of precursors with SP or TMH into the Sec61 channel and the concomitant gating of the Sec61 channel to the open conformation occur spontaneously or involve client-specific auxiliary components of the Sec61 channel (TRAP, Sec62/Sec63 complex) (
Figure 1 and
Figure 3). Typically, the orientation of SP- and TMH in the Sec61 channel follows the positive inside rule; i.e., positively charged amino acid residues in the N-region support loop insertion (N
in-C
out) and positively charged residues downstream of the SP or TMH interfere with loop insertion and, therefore, favor head-on insertion (N
out-C
in) into the Sec61 channel (
Figure 2)
[117][118][119][120]. Next, TMDs can enter the phospholipid bilayer via the lateral gate of the fully open Sec61 channel by lateral movement and large hydrophilic domains (i.e., with more than 50 amino acid residues), or entire soluble proteins can be translocated into the ER lumen by vectorial movement through the channel (
Figure 1 and
Figure 2). Alternatively, membrane insertion of some precursors of MPs can be facilitated co- or posttranslationally by evolutionarily conserved MP insertases that comprise a hydrophilic vestibule near the cytosolic surface of the ER membrane, but cannot form an aqueous channel through the ER membrane, and, therefore, cannot translocate hydrophilic domains with more than 50 amino acid residues across the ER membrane. These Oxa1-related insertases are the Wrb/Caml complex (in co-operation with cytosolic GET3 of the abovementioned TRC pathway), or the multimeric ER membrane protein complex (EMC, which may occasionally co-operate with the Sec61 complex), or the GEL-BOS-PAT complex, which depends on the interaction with but not the channel activity of the Sec61 complex (
Figure 3)
[121][122][123][124][125][126][127][128][129][130][131][132]. Notably, the first has its exclusive role in the membrane insertion of TA membrane proteins
[92][95][98]. In the case of the EMC, a proteomic approach identified TA proteins, as well as multispanning MPs, as the predominant clients, the latter of which were also characterized as the main clients of the GEL-BOS-PAT-Sec61 supercomplex but proposed to have a preference for multispanning MPs with four or more TMDs, including those with marginal hydrophobicity
[131][132]. There are two excellent reviews, plus several very recent original articles, on the structural and mechanistic details of the various MP insertases in the ER membrane
[69][70][81][121][122][123][124][125][126][127][128][129][130][131][132].
5. A Single Proteomic Approach to Address the Client Spectra of Various Components for Targeting of Precursor Polypeptides to and Insertion into the Human ER Membrane
Following the pioneering work by G. Blobel and B. Dobberstein
[13][14], protein import into the human ER was usually studied with the focus on single precursor polypeptides that were analyzed one-by-one in cell-free assays or intact cells. These classical studies led to the conclusions of whether and how the targeting and membrane insertion or translocation of a certain precursor is facilitated by a certain component. Recently, more global approaches were developed, such as the already mentioned proximity-specific ribosome-profiling
[29][111][126][133][134] and quantitative proteomics approaches
[5][106][112][126][135][136][137][138][139]. Typically, the proteomic approach employed siRNA-mediated knock-down or CRISPR/Cas9-mediated knock-out of components one-by-one in human cells, label-free quantitative proteomic analysis, and differential protein abundance analysis to characterize client specificities of components (
Figure 4, left part). In contrast to the classical analyses, the quantitative proteomics approach addresses the question of which precursors use a certain pathway or component in intact cells, i.e., under in-vivo-like conditions (
Figure 4, right part).
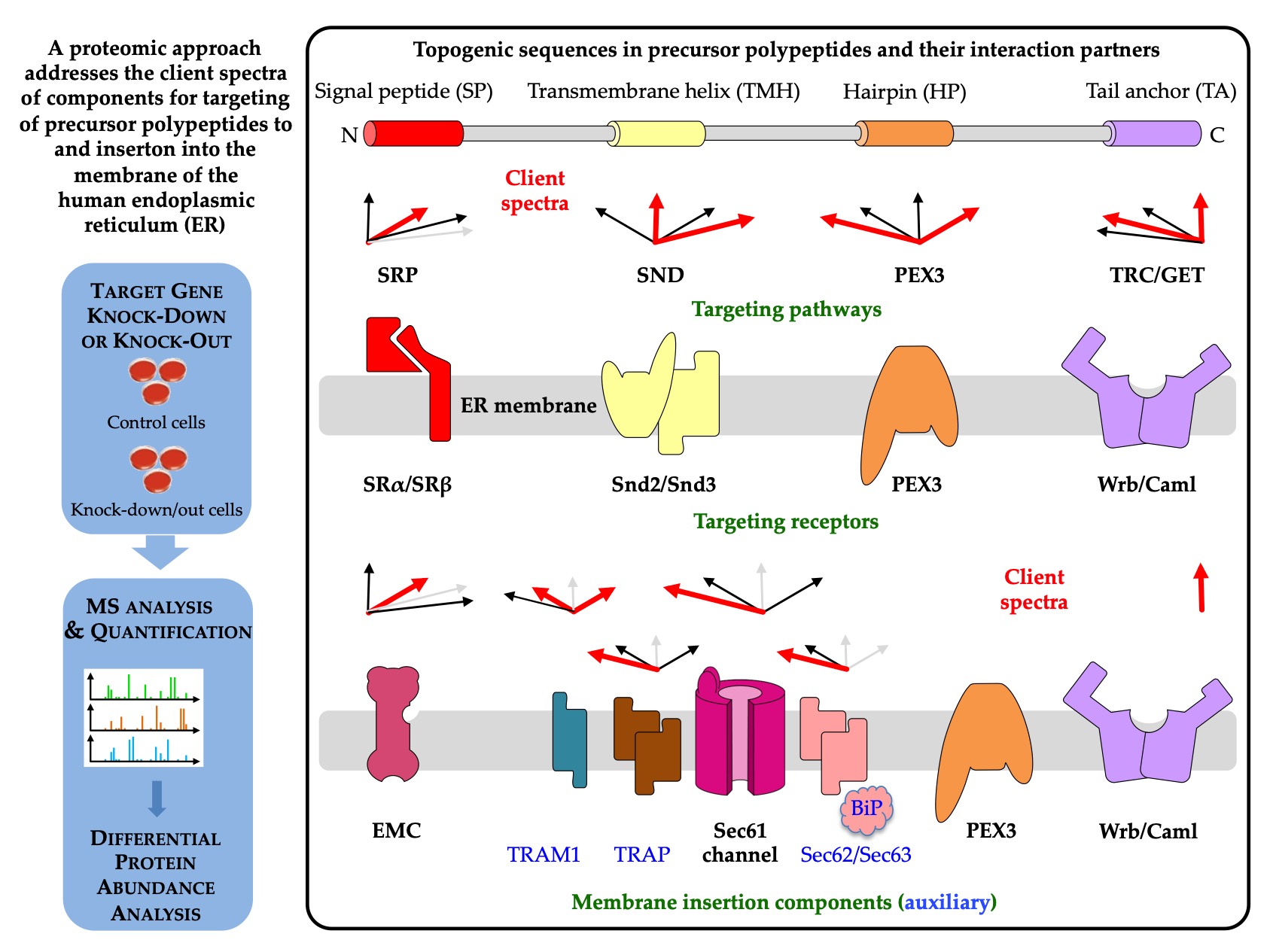
Figure 4. Components for protein targeting to and insertion into the human ER membrane with their client spectra and possible regulatory mechanisms. The first two percentages in each client spectrum refer to the percentage of precursors with SP and TMH (including HP and TA proteins), respectively, among the total number of clients, while the following two numbers refer to the percentage of precursors with HP or TA among the precursors with TMH. The red arrows highlight top scoring numbers > 10, light grey arrows represent the number 0 and are shown to indicate that fact.