The GBM microenvironment is a dynamic entity comprised of several different types of cells and factors, which include tumor cells, GSCs, endothelial cells, astrocytes, microglia, stromal components, soluble factors, extracellular matrix, and other substances
[9][18]. Each component plays an important and specific role in the microenvironment. It has been shown that GSCs, for example, can establish a continuous cross-talk that actively assists in the remodeling of the microenvironment. These factors can be influential in maintaining and contributing to the progression of the disease
[6][8][9][11][12]. Identifying the key actors in this scenario has become increasingly challenging, as the dialogue between GSCs and the microenvironment plays a significant role in supporting GBM
[9][10][11][12][13][14][15][16]. The TME also includes immune and myeloid cells. Myeloid cells are the most frequent and, among them, tumor-associated macrophages (TAMs) are the most abundant
[3][6][14][17][19][20].
TAMs are the major biological constituents of the GBM TME and are implicated in GBM progression, angiogenesis, and, according to recent reports, the development of resistance to adjuvant treatments
[3][5][6]. GBM cells and TAMs are constantly in close cross-talk, communicating through paracrine signals, including cytokines and the extracellular production and release of exosomes, which are a novel class of extracellular vesicles that have gained enormous attention lately as facilitators of the progression of various tumors
[18]. Exosomes are secreted by different cells in the body, including tumor cells and other cells in the tumor microenvironment, and are mainly used as vehicles to exchange information between cells. Their content in terms of nucleic acids (mainly DNA, mRNA, and miRNA) is dynamic and related to the cells of origin. Since each miRNA is responsible for the regulation of several mRNAs, the transport of different miRNAs from one cell to another via exosomes involves the regulation of several mRNAs in the acceptor cell and, as the final outcome, modifies its behavior. The exchange of information that follows the release of exosomes is capable of mutually modifying the phenotype of tumor cells and those in the tumor microenvironment. miRNAs and lncRNA can be transmitted via exosomes from cancer cells to other cells in the TME and vice versa, influencing tumor development, aggressiveness, and progression and TAM immunosuppressive polarization
[18][21][22][23][24][25][26].
It has been currently postulated that TMZ resistance in GBM can be reflected by extracellular vesicles. Yin et al.
[15] found that high serum levels of exosomes from GBM patients containing miRNA-1238 are indicative of TMZ resistance of the GBM cells. Chuang et al.
[27] provided evidence that miRNA-21 and miR-416a enriched TAM M2-derived exosomes, contributing to GBM malignancy via increasing stemness and favoring the development of TMZ resistance. Witusik-Perkowska et al.
[28] showed that miRNA-31, miRNA-221, miRNA-222, and miRNA-21 are expressed at higher levels in the serum of GBM patients with TMZ resistance. These miRNAs, downregulating PTEN expression in tumor cells, may reduce the sensitivity of GBM cells to TMZ. All this evidence leads to the assumption that specific miRNAs’ exosomal profiles (miRNA-31, miRNA-21, miRNA-221, miRNA-416a, miRNA-222, and miRNA-1238) in GBM patients’ serum could be considered as a possible biomarker in developing CTX protocols. Additional clinical data and studies, however, are needed to develop efficient, reliable, cost-effective, and convenient methods for identifying circulating miRNAs
[15][18][21][22][23][24][25][26][27][28].
The role of miRNAs in favoring chemoresistance has been documented in current literature, however, there is less evidence regarding the role of lncRNAs. Wu et al.
[29] demonstrated that lnc-RNA TALC could regulate M2 polarization and promote TMZ resistance in GBM by activating the p38 MAPK signaling pathway and promoting C5a release. In addition, Zhang et al.
[23] demonstrated that exosome-mediated transfer of lncRNA 226 SBF2-AS1 from TAMs spreads TMZ resistance in GBM cells through a mechanism of upregulation of serum SBF2-AS1 levels, mediated by the transcription factor ZEB1, which binds directly to the SBF2-AS1 promoter region. Dai et al.
[13] and Zheng et al.
[30] showed that AHIF and lncRNAs linc-RA1 may contribute to GBM radioresistance. In detail, AHIF mediated radioresistance through VEGF-A and angiogenin in secreted exosomes
[13]. On the other hand, lincRA1 stabilized the level of H2B K120 monoubiquitination (H2Bub1) by combining with H2B and inhibiting the interaction between H2Bub1 and ubiquitin-specific protease 44 (USP44), which inhibited autophagy, thus contributing to GBM radioresistance
[30].
By the same mechanism, lnc-TALC also appears to favor the development of GBM radioresistance. The lncRNAs do not seem to play a role only in the development of TMZ resistance; they may also contribute to the development of GBM radioresistance. Zhang et al.
[23] demonstrated that exosome-mediated transfer of lncRNA SBF2-AS1 from TAMs spreads TMZ resistance in GBM cells, while Dai et al.
[13] and Zheng et al.
[30] showed that lncRNAs linc-RA1 and AHIF may contribute to GBM radioresistance. This evidence suggests that the blocking of lncRNA-mediated cross-talk between GBM cells and TMAs might be a novel therapeutic strategy to address TMZ resistance and radioresistance. Moreover, specific lncRNA identifiers of an aggressive TME, predisposing to the development of TMZ resistance, can be searched for in the blood, as can miRNAs, as a biomarker of diagnosis of GBM and prognostic factor of adjuvant resistance therapies
[13][23][29][30].
TAMs can be categorized into two subtypes based on their function: M1 and M2 polarized macrophages. Studies have linked M2 macrophages to increased GBM aggressiveness by secreting various molecules, such as PDGF, EGF, TGF- β1, and VEGF, to surrounding cells. However, as shown more recently, M2 macrophages may also promote the TMZ resistance and radioresistance of GBM cells
[8][11][16][26][31][32]. Recent data suggest that M2 macrophages may play an important role in regulating TMZ resistance. Zhang et al.
[5] and Azambuja et al.
[33] showed that VEGF-dependent M2 macrophages can activate the PI3K/Akt/Nrf2 pathway to favor TMZ resistance in individuals with GBM. These results agree with previous reports that showed that upregulated VEGF levels can be important in TMZ resistance. Chuang et al.
[27] showed that eliminating M2 macrophage-derived miRNA-21 exosomes can overcome GB’s TMZ resistance. M2 macrophages have been shown to have the typical surface biomarker CD163. Miyazaki et al. reported that CD163-positive M2 macrophages could also play a role in the increased immune therapy resistance in GBM cells that show TMZ resistance
[34].
Accordingly, M2-polarized macrophages promote cancer stemness and chemoresistance in other types of tumors, including pancreatic cancer and thyroid cancer. Thus, in light of the numerous data reported in the literature, it is reasonable to speculate that M2 macrophages may contribute to cancer stemness, progression, aggressiveness, and TMZ resistance in GBM
[35][36].
M2 macrophages appear to have a pivotal role also in the development of GBM radioresistance. Jang et al.
[37] reported that the M1/M2 macrophage ratio in the TME and radiosensitivity of GBM cells are inversely associated. In this regard, short-term relapse GBMs had a significantly higher fraction of M2 macrophages after RT compared with the long-term relapse tumors detected, suggesting that M2 macrophages may play a role in radioresistance and then develop an early relapse. Furthermore, several studies have suggested that immunotherapy targeting M2 macrophages may favor the radiosensitivity of GBM cells
[33][38]. These first findings highlight the primary role of M2 macrophages in the development of GBM radioresistance. The increase of the M1/M2 ratio by conversion of M2 macrophages into M1 macrophages or the destruction of M2 macrophages may, thus, represent a potential therapeutic approach for increasing GBM radiosensitivity
[26][31][32][33][34][39][40].
To summarize, a high proportion of M2 macrophages’ and specific miRNAs’ (including miR-1238, miRNA-31, miRNA-221, miRNA-222, miRNA-416a, and miRNA-21) and lncRNAs (lnc-TALC, SBF2-AS1, linc-RA1, and AHIF) profiles in the TME may play a pivotal role in the acquisition of TMZ resistance and radioresistance of GBM cells. Exosomal miRNA and lncRNA levels in human serum may function not only as a potential diagnostic biomarker in GBM patients, but also as a prognostic factor for the early identification of TMZ resistant and radioresistant GBMs
[31][33][34][39].
Macrophage-targeting immunotherapy has been proposed as a potential tool to improve GBM treatment strategies by preventing TAMs recruitment, repolarizing TAMs, and using immune checkpoint blockade
[41]. M2-like phenotype TAMs tend to be important in the immunosuppressive TME, which can secrete immunosuppressive substances like TGF-b, IL-6, and IL-10 in GBM. The secreted pro-inflammatory cytokines include IL-12, IL-2, IFN-g, and TNF-a, which can be detected in low levels
[42][43]. The M1 to M2 phenotype transition in TAMs tends to be associated with the progression of the tumor. TAMs have been shown to be important in the progression of GBM by pro-tumorigenic activities, which include GBM cell migration, proliferation, and invasion. TAMs have also been shown to play an important role in the activation of angiogenesis, generating immunosuppressive TME, and facilitating the degradation of the extracellular matrix (
Figure 1)
[44][45].
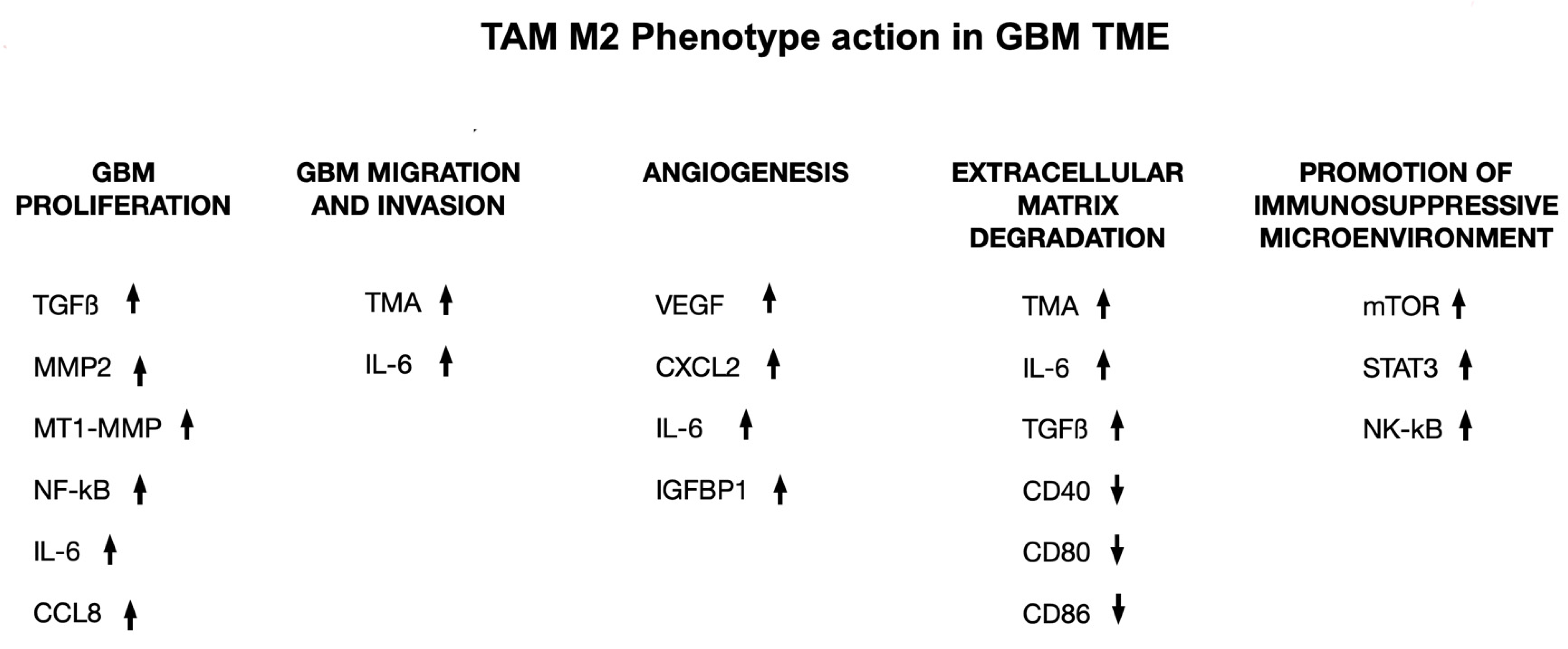
Several TAM substances, like stress-inducible protein (STI)-1, TGF-β, IL-1β, IL-6, and EGF, have been shown to enhance GBM cell invasion. Recent studies have shown that M2 macrophages play a role in promoting the vascularization of brain tumors by secreting pro-angiogenic factors like VEGF. As a result, TAM pathways have emerged as promising targets for immunotherapy. In vitro and in vivo analyses have shown that targeting these pathways can lead to reduced myeloid infiltrates, decreased tumor vascularity, and improved overall survival
[45][46].
Accordingly, over the years, macrophage-targeting immunotherapy has been advocated for as a potential tool to improve GBM treatment strategies. In detail, three of the main macrophage-targeting immunotherapy strategies include: TAMs recruitment prevention, TAMs repolarization, and immune checkpoint blockade.
An effective strategy for treating GBM may involve preventing the recruitment of M2 macrophages to the GBM site, considering their role in tumor invasion and progression. Ongoing clinical trials are targeting this approach with inhibitors for the following targets: angiopoietin-2 (ANG2), CXCR4, and colony-stimulating factor 1 receptor (CSF1R),
[47][48][49][50][51]. Another potential strategy is promoting a shift between M2 and M1 macrophages. CD40 and TLR agonists are currently being tested in GBM clinical trials as potential therapeutic agents targeting TAM repolarization
[52][53][54][55]. In addition, immune checkpoint inhibitors such as anti-PD-1 and anti-CTLA-4 have shown success in treating melanoma and non-small cell lung cancer in recent years. Therefore, immune checkpoint blockade is a promising treatment that is also implicated in clinical trials for GBM (
Table 1)
[56][57].
Table 1. Review of clinical trials on immunotherapy targeting GAM in GBM (from ClinicalTrials.gov).
3. Future Perspectives
GAMs are associated with the radio-obstruction of glioblastoma by discharging TNF α, which increments the atomic element κB (NF-κB) that is connected with substandard endurance. Different techniques have been considered to lessen the enlistment of GAMs in GBM movement, including adjusting the GAMs aggregate towards an enemy of growth M1-like microenvironment, or diminishing the M2-like cancer-advancing microenvironment (11). For GBM treatment, TLRs (toll-like receptors) could be new targets. They suppress pro-tumorigenic pathways by modulating immune responses in GBM and the innate immune system. Hence, they could address a possible new objective in GBM treatment. Polyinosinic-polycytidylic acid and poly-L-lysine (Poly ICLC—Hiltonol), a TLR3 agonist, can activate immune cells and encourage their migration into the tumor mass. In a stage I preliminary, recently analyzed GBM patients were given Poly ICLC in blend with standard consideration and customized peptides, in light of individual cancer transformations, as a growth explicit antigen immunization. Other immunostimulants, such as the TLR3 agonist Poly I, were also tested with Poly ICLC: C and TLR7/8 agonist Imiquimod in malignant growth antibody treatments on patients who went through complete growth resection of GBMs. These treatments increased OS and progression-free survival (PFS), indicating potential for future treatments
[58].