The windfall for human and animal health in terms of effectively fighting infectious diseases is being threatened by the resurgence and appearance of dangerous pathogens, which largely outpace the discovery and implementation of new antimicrobials. Growing resistance to current antibiotics
[1], antifungals
[2], and antivirals
[3] is one of the greatest reasons for this. This situation is substantially worsened by the long-lasting drought (with a few recent exceptions
[4]) in the discovery of new classes of antibiotics since 1962
[1][5] and the continued scarcity and specificity of antifungals
[6] and antivirals
[7]. On top of that, factors including population growth, intensive farming, globalization, pollution, and climate change are also contributing notably to this issue by negatively unbalancing the pathogen–host–environment interplay
[8].
Thus, in order to address the public health menace posed by both new and “renewed” infectious diseases that are quite often unfortunately associated with considerable morbidity and mortality, it is crucial to expand the arsenal of antimicrobials. This is because, even in an unfavourable scenario of rapid generation of antimicrobial resistance, their availability would at least help to buy time for the development of other countermeasures, such as effective vaccines. In this regard, different antimicrobial search and development strategies with high expectations are being adopted
[9][10][11].
2. Fish Skin Mucus as a Promising Source of Antimicrobials
In this context, marine ecosystems still remain an option with great potential for the discovery of new compounds, as they are relatively unexplored in this regard. Furthermore, they are the most extensive and ecologically diverse ecosystems, and, therefore, harbor the largest biological and hence biochemical diversity on the planet
[12][13][14][15]. As expected, in this highly competitive environment
[12][16], microorganisms are currently the fastest growing group of marine producers from which new compounds and antimicrobials are being discovered
[17][18].
However, the contribution of higher counterparts, such as algae, plants, and animals, has been, and still is, particularly important
[17][19], even considering that many compounds initially attributed to them may actually belong to associated or symbiotic microorganisms
[17][20]. Among the animals, the majority of suppliers are invertebrates, mainly (in order of contribution) sponges as the overall top producer of marine natural compounds so far, molluscs, tunicates, coelenterates, echinoderms, and bryozoans
[17][19]. In this ranking, the contribution of marine vertebrates, almost entirely represented by fish, is still rather modest (just right after coelenterates)
[17][18], but certain factors, which will be commented on next, encourage further research into the potential antimicrobials that they may offer, especially from their skin mucus
[21][22][23].
Marine ecosystems are regulated by complex interactive fluxes that are primarily controlled by microorganisms due to the predominance of their biomass
[24]. With a focus on viruses, bacteria, and fungi, some quantitative studies have estimated the abundance of each of the first two groups in the millions per milliliter of seawater
[16][25][26]. The virus is the predominant microorganism in the ocean, accounting for about 10
30 particles, about 15 times more than estimated bacteria (and archaea)
[16]. There is little information on the quantitative abundance of fungi in aquatic environments, although it is assumed to be relatively high based on data on their enzymatic activity in certain environments compared to bacteria
[27].
As a result, fish have co-evolved under this selective pressure by also developing a complex network of defense mechanisms, such as the adaptive immune system
[28][29][30]. However, although they have one of the earliest forms of adaptive immunity, their innate immunity still plays a central role in protecting them from and responding to infection
[28][31], especially through a complex system of mucosal barriers responsible for fending off pathogens on first contact
[28][31][32]. In fact, leukocyte distribution in fish is more organized in the mucosal tissues of the gut, gills, and skin than in the liver or gonads, for example
[28][32]. Besides the cellular immune component, the humoral aspect of these tissues is of special relevance because of its antimicrobial function
[33]. Among these major mucosa-associated lymphoid tissues (MALT), i.e., gut (GALT), gills (GIALT), and skin (SALT), mucosal glands are much more numerous in the skin
[31][34], which is reasonable considering its continuous and intimate exposure to large amounts of microorganisms
[16][25][26][27].
3. Composition of Fish Skin Mucus in Innate Immunity Antimicrobial Molecules
3.1. Antimicrobial Peptides (AMPs)
AMPs, also known as Host Defense Peptides (HDPs), are gene-encoded peptides of up to approximately 80 amino acid residues, mostly characterized by a cationic, amphipathic chemical nature and antimicrobial properties. They are ancient innate immune molecules present in all groups of organisms. Their mature forms in eukaryotic cells are often cysteine-rich molecules with multiple intramolecular disulfide bridges. Through conservation or reduction of these bonds, some families of AMPs can modulate their type and/or level of activity
[35][36][37][38]. For instance, in defensins (one of the most studied families of AMPs), some reports on a particular group of human beta-defensins indicate that the reduction of such bonds affects their function by disabling their chemotactic activities and triggering their direct antimicrobial ones
[37].
Given the importance of these molecules in the innate immune system, they are extremely diverse in fish and include not only families of AMPs found in other animal groups, such as cathelicidins, defensins, hepcidins, and histone-derived peptides, but also exclusive fish AMP families, such as piscidins and pleurocidins
[39][40]. Probably also for this reason, the skin mucus is the major source of AMPs in fish, with approximately 70% of all AMPs expressed in the skin compared to 52% and 29% expressed in the gills and the gut, respectively
[31][34]. Besides expression, several AMPs were isolated from skin mucus, and their antimicrobial activities were tested.
Histone-derived AMPs were first described in fish
[41] just a few years after their discovery in the Asian toad
Bufo bufo gargarizans [42]. Robinette et al., (1998)
[41] isolated two histone-like proteins (HLP-1 and HLP-2) in the epidermis of channel catfish that were found to be inhibitory to bacterial and fungal pathogens. Shortly thereafter, several histone-derived peptides were isolated from fish skin mucus
[43][44][45][46]. In general, this family of AMPs is thought to be released from cells during infection-induced apoptosis
[47]. Oncorhyncin III is a 66-residue N-terminal fragment of the non-histone chromosomal protein H6 from
O. mykiss skin mucus and was shown to be active against gram-negative and gram-positive bacteria
[48].
Other peptides found in fish skin mucus include myxinidin, pardaxin, pelteobagrin, and piscidin, all of which are unique to this group of animals and have been reported to have broad-spectrum antimicrobial activity. Myxinidin is a cationic 12-amino acid peptide isolated from the skin mucus of hagfish (
M. glutinosa)
[49]. Pardaxin was first isolated from the Red Sea Moses sole (
P. marmoratus) and described as a single, helical, monomeric, acidic toxin
[50]. Its antibacterial activity against gram-negative and gram-positive bacteria was subsequently demonstrated
[51]. Pelteobagrin is a 20-amino acid amphipathic α-helical peptide and was identified in the skin mucus of yellow catfish (
P. fulvidraco)
[52]. The piscidin family also comprises α-helical peptides, with low molecular weight and cationic charge at physiological pH
[53].
3.2. Proteins
Animal mucosa, in a broad sense, is characterized by the presence of mucins, which are glycosylated proteins responsible for providing viscoelastic and rheological properties, as well as trapping pathogens and contributing to cell surface signaling. Other types of glycoproteins have been found in fish skin mucus. For example, Ebran et al., (2000) [54] isolated and characterized glycoproteins from rainbow trout (Oncorhynchus mykiss), European eel (Anguilla anguilla), and tench (Tinca tinca) skin mucus. These proteins possess both α-helix and random coil structures and show antibacterial activity correlated with pore-forming properties. Transferrin glycoprotein has also been isolated from Atlantic cod [55] and Atlantic salmon (Salmo salar) [56] skin mucus. Transferrin is responsible for iron transporting in absorption, storage, and disposal sites in vertebrates.
Lectins are a diverse class of highly specific carbohydrate-binding proteins [57]. They have been found in the skin mucus of fish, where they provide an external defense mechanism via the agglutination process to stop pathogen penetration and colonization [58]. There are several types of different lectins depending on their structure; for example, C-type lectins, whose binding is dependent on Ca2+, F-type lectins or fucolectins, which are distinguished by their α-l-fucose recognition domain, galectin family or S-type, which require thiol, and pentraxins or pentameric lectins, or P-type lectins, which target glycoproteins containing mannose 6-phosphate [57]. The isolation of C-type lectins has been described in cichlid (Symphysodon aequifasciata) skin mucus [59].
Lysozyme (N-acetylmuramide glucanohydrolase or muramidase) is a bacteriolytic enzyme and an important component of the immune system. It has been reported in the skin mucus of several fish species, including mrigal carp (
Cirrhinus mrigala), catla (
Catla catla), spotted snakehead (
Channa punctata), Japanese eel (
Anguilla japonica), and Nile tilapia (
Oreochromis niloticus)
[60][61][62]. Given its ability to hydrolyze the bond between N-acetylmuramic acid and 3-acetyl amino-2-deoxy-D-glucose residues of the mucopolysaccharide found in bacterial cell walls
[63], it acts directly on gram-positive bacteria. In gram-negative bacteria, lysozyme can also attack the inner peptidoglycan layer after the disruption of the outer wall by complement and other enzymes
[61].
Proteases are enzymes of great importance in the mechanisms of the immune system. Their role is to hydrolyze the peptide bonds of proteins. Proteases can be classified into serine, cysteine, aspartic, and metalloproteases based on their catalytic mechanisms
[64]. They are associated with resistance to infection because of their ability to degrade the proteins of pathogens. Proteases, including trypsin (serine protease), cathepsin B and L (cysteine proteases), cathepsin D (aspartic protease), aminopeptidases, and metalloproteases, have been reported in the skin mucus of several species, such as rainbow trout
[65], Japanese eel
[66], European eel
[67], catfish (
Parasilurus asotus)
[46], and Atlantic salmon
[68].
3.3. Other Components
The lipid composition of fish skin mucus has not been studied as thoroughly as other mucous secretions, such as gut mucus. However, some studies show that skin mucus may also be a significant source of lipids. Mono-unsaturated fatty acids (MUFA), such as oleic acid, poly-unsaturated fatty acids (PUFA), such as linoleic, alpha-linoleic, docosahexaenoic, arachidonic, eicosapentaenoic, and moroctic acid, and saturated fatty acids (SFA), such as palmitic and stearic acid, have been reported in gilthead sea bream and flathead grey mullet (
Mugil cephalus)
[69][70]. Lipids are thought to be involved in maintaining the internal structure of the mucus through interactions with glycoproteins
[71].
4. Antimicrobial Activity of Fish Skin Mucus
The humoral component of fish skin mucus has been extensively studied for its high content in molecules endowed with antimicrobial properties and, thus, its potential for implementation in biomedical and veterinary applications. Such a variety of compounds has also necessitated the use of different molecular extraction approaches in mucus samples (Figure 1).
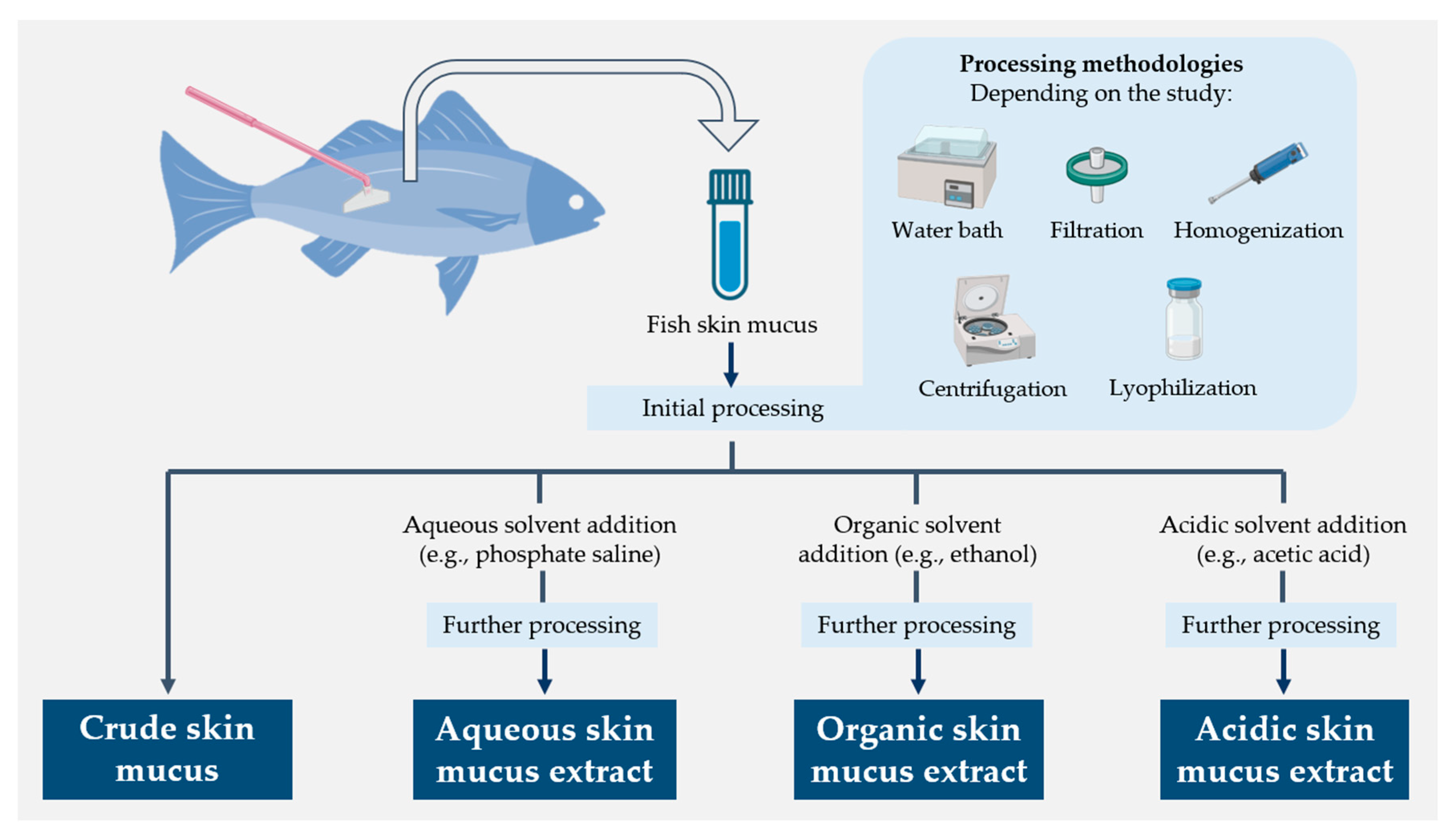
Figure 1. Schematic diagram summarizing the different molecular extraction approaches used for fish skin mucus samples
4.1. Antibacterial Activity of Fish Skin Mucus Extracts
4.1.1. Aqueous Extractions
The most frequently used extraction method was the aqueous one. The most commonly employed solvents in these studies, listed in order of frequency of use, were physiological saline, water, ammonium bicarbonate, and Tris-buffered saline.
Although aqueous extraction was the most popular extraction method, it also showed the least antibacterial activity. This was particularly evident in those experiments where different extraction methods were compared. In some experiments, aqueous extracts did not show any antibacterial activity [72][73][74][75][76][77][78]. For example, Subramanian et al., (2008) [76] found antimicrobial agents, such as lysozyme, cathepsin B, and trypsin-like proteases, in the aqueous skin mucus extracts of several fish species, but they did not exert any antimicrobial activity. Al-Rashed et al., (2018) [72] used aqueous and acidic extracts of the skin mucus of the climbing perch (Anabas testudineus), but only found antibacterial activity in the latter. Similarly, Hellio et al., (2002) [74] performed aqueous and organic extractions of the skin mucus of the ballan wrasse (L. bergylta), but only observed antibacterial activity with the organic extracts. Subhashini et al., (2013) [78] did not find antimicrobial activity in the aqueous extract of the skin mucus of tinfoil barb fish (Barbonymus Schwanenfeldii), even if the amount of protein was higher than in the organic extracts obtained in parallel.
4.1.2. Organic Extractions
For the organic extractions, the most used solvents have been ethanol and dichloromethane. Some authors performed alcoholic extractions and then partitioned distilled water with dichloromethane to obtain aqueous and organic phases [74][78]. High antibacterial activity has been reported for organic fish skin mucus extracts. Indeed, in some studies, all bacteria tested were inhibited, both gram-positive and gram-negative [74][78][79][80]. The main reasons explaining such activity are that (i) the presence of hydrophobic groups is often a common feature of antimicrobial molecules because of their affinity for membranes and their ability to disrupt them [81]; and (ii) these extracts are enriched in hydrophobic molecules because organic solvents favor their isolation by reducing the interactions between hydrophobic groups, which hinders their aggregation [82]. In fact, Mahadevan et al., (2019) [80] obtained greater inhibitory activity against gram-negative and gram-positive bacteria using organic mucus extracts compared to aqueous ones. Hellio et al., (2002) [74] correlated high antimicrobial activity with low polarity of the solvents used; they also showed that extracts from the dichloromethane phase were more active than those from the aqueous phase. In a study by Bergsson et al., (2005) [79], an organic (acetonitrile (ACN) + 1% trifluoroacetic acid (TFA)) extract of cod skin mucus exhibited high antimicrobial activity against gram-positive and gram-negative bacteria. In these extracts, they also identified four peptides with known antimicrobial activity, i.e., those derived from the histone H2B and the 60S ribosomal proteins L40, L36A, and L35.
4.1.3. Acidic Extractions
For acidic extractions, the most common solvent was acetic acid (AA) followed by TFA. In general, acidic extracts showed greater antibacterial activity than other extracts
[72][73][76][77][78][83][84][85][86]. In most studies using acidic extractions to determine antibacterial capacity, all bacteria tested were inhibited
[73][76][83][84][85][87]. This may be due to the presence of cationic peptides and defensive low-molecular-weight proteins. This type of molecule has been shown to be more soluble in mildly acidic solutions
[88].
4.1.4. Crude Mucus
Finally, some studies have evaluated the activity of fish skin mucus in its almost raw form, without any type of solvent extraction. Sanahuja et al., (2019)
[89] compared the crude skin mucus of gilthead sea bream, European sea bass, and meagre (
Argyrosomus regius). In particular, meagre mucus showed biocidal activity against all bacterial species tested, i.e.,
E. coli,
V. anguillarum, and
P. anguilliseptica (all gram-negative), which was associated with higher levels of non-specific defenses, such as protease and carboxylesterase activities. Fuochi et al., (2017)
[90] studied the antibacterial activity of common stingray (
D. pastinaca) crude skin mucus and found that it inhibits the bacterial growth of gram-negative, but not gram-positive, bacteria. This observation was attributed to a strong interaction between the outer membrane (present in gram-negatives only) and the biomolecules present in the mucus. They also demonstrated the presence of chitinase 1, an enzyme involved in the degradation of chitin
[90].
4.2. Antifungal Activity of Fish Skin Mucus Extracts
Several studies have produced mixed results using crude fish skin mucus. On the one hand, the antifungal activity of crude skin mucus of catla, mrigal carp, and European eel inhibited the growth of Aspergillus awamori, Colletotrichum falcatum, and Fusarium oxysporum in the study by Pethkar et al., (2017) [91]. Fuochi et al., (2017) [90] also found that the crude skin mucus of the common stingray was active against Candida albicans, Candida glabrata, and Candida tropicalis. On the other hand, Hisar et al., (2014) [92] tested the crude skin mucus of rainbow trout against C. albicans and Candida parapsilosis, but no antifungal activity was observed. Ikram et al., (2013) [93] screened the antifungal activity of crude and aqueous (i.e., PBS and water) skin mucus of Asian swamp eel (Monopterus albus) against C. albicans, Candida krusei, Cryptococcus neoformans, and Fusarium spp., but only the water extract revealed an inhibitory effect, with activity against all the fungi tested and mostly against Fusarium spp.
4.3. Antiviral Activity of Fish Skin Mucus
Information on the antiviral activity of fish skin mucus extracts is scarce to date. Raj et al., (2011)
[94] investigated the role of carp epidermal mucus as an innate immune barrier against CyHV-3 entry. They found that skin mucus inhibits CyHV-3 binding on epidermal cells and leads to a significant reduction in the number of viral plaques. This reduction only occurred when cells were pre-incubated with mucus, but not when mucus was added after the incubation period. Most of the studies, however, report antiviral activity of compounds that had been previously isolated from skin mucus. For instance, Valero et al., (2020)
[95] reported the presence of NK-lysin in Atlantic salmon skin mucus, and Falco et al., (2019)
[96] demonstrated its antiviral activity against spring viremia of carp virus (SVCV) by inhibiting not only the binding of viral particles to host cells, but also the fusion of virus and cell membranes. Beta-defensins, an important factor in the antimicrobial barrier function of the skin
[97], have also been shown to have antiviral activity against another rhabdovirus, the viral haemorrhagic septicaemia virus (VHSV)
[98].
5. Omics Techniques as a Promising Tool in Fish Skin Mucus Research
The field of fish skin mucus research has generally been limited by the complexity of its composition, as well as the interactions between its components. Omic techniques have recently emerged to provide a holistic approach to cellular components and their interactions, providing an effective tool towards a deeper understanding of marine systems
[99]. The progress of these techniques has allowed the field of studies of fish skin mucus to grow quickly in recent years
[21]. These techniques have been applied to fish skin mucus research in different topics, such as welfare, health, and nutrition. However, the discovery of antimicrobial agents through these methods is an underexplored opportunity.
Genomics have provided insights into molecular and genetic mechanisms in fish. Ao et al., (2015)
[100] sequenced and assembled the genome of
Larimichthys crocea using a bacterial artificial chromosome and a whole-genome shotgun hierarchical strategy. They identified 159 genes related to mucin biosynthesis and mucus production based on previous studies in mammals, thus suggesting that the mucin synthetic pathway is conserved between fish and mammals.
Transcriptomics provides information on the RNA transcripts produced by the genome, from protein coding (mRNA) to noncoding RNA. A recent study examined the efficacy of whole-transcriptomic profiling of mahi-mahi epidermal mucus as a method for oil exposure detection using RNASeq
[101]. Transcripts involved in immune response, cardiotoxicity, and calcium homeostasis showed differential expression after oil exposure, which indicates that mucus is a promising source for noninvasive monitoring techniques.
Proteomics is the characterization of proteins expressed in an organism. It is the most studied omic technique in fish mucus research. Proteomics provides information about the entire effect of the gene expression process and encompasses post-transcriptional and post-translational protein expression regulation
[102]. Proteomic profiles of the skin mucus of gilthead seabream
[103], Atlantic cod
[104], Atlantic salmon
[105], mudskipper
[106], discus fish (
Symphysodon spp.)
[107], European sea bass
[108], and lumpsucker
[109] have been published, allowing for the possibility of comparative studies to better understand the dynamics of fish mucus. Moreover, the proteomic profile of fish mucus subjected to different types of stress has been studied, such as chronic wounds
[110], bacterial infection
[111][112], parasitic infection
[113], artificial stressors
[114], and sample collection
[115]. These types of studies expand our knowledge of proteomic changes associated with immune processes, and they can be a starting point to develop a powerful tool to identify bioindicators of fish welfare and physiological status via non-invasive methods.
Metabolomics can be defined as the quantitative complement of all low-molecular-weight molecules present in cells in a particular physiological or developmental state
[116]. Metabolomics is situated downstream of proteomics, transcriptomics, and genomics, thus making metabolomics extremely useful for understanding organism responses and for biomarker discovery
[22]. Analytical methods in metabolomics commonly include mass spectrometry (MS), often in conjunction with gas chromatography (GC) and liquid chromatography (LC), and nuclear magnetic resonance (NMR). Studies on fish metabolomics cover a wide range of fields of knowledge, including fish physiology and development, pollutants’ effects on fish, fish condition and disease, and fish as foodstuff
[117].
6. Conclusion
Besides being a key component in several physiological functions, fish skin mucus provides an effective chemical and physical barrier against pathogens. The performance of this activity is highly dependent on mucus composition. Therefore, the choice of a suitable molecular extraction method is crucial for its antimicrobial use in other applications. Indeed, notable differences in antimicrobial activity have been shown for the different types of extracts, which is particularly relevant in those studies comparing different extraction methods on the same samples. In general, acidic extracts, followed by organic ones, showed the highest antimicrobial activity. This may be because these procedures favor the isolation of cationic and/or amphipathic antimicrobial compounds, such as AMPs, their enrichment in the final extracts and, apparently, the minimization of molecular inactivation events.
7. Recommendations and Future Perspectives
This entry emphasizes the importance of mucus composition for its antimicrobial activity. However, this composition may differ notably depending on several factors, such as species, sex, age, and environment. Therefore, more information on these factors should be included in such studies to improve reproducibility. A practical option could be to focus these studies on animals at harvesting stages in order to normalize the results at the most appropriate time for industrial exploitation. In this sense, it would also be of great interest to develop efficient technologies for the collection of fish skin mucus at harvesting sites.
However, the momentum needed to accelerate progress in these lines of research and their translation into practice requires a substantial increase in the need for these products. The urgency for new antimicrobials noted in the introduction is one such need. However, new ideas that define the targets against which these compounds could already make a difference would greatly accelerate their development. Examples include the use of marine antimicrobials in high ionic strength environments, such as the mucosal tissues of cystic fibrosis patients or food preservation. In this line of research, it would also be interesting to study formats to increase their stability and to improve their delivery; for example, by encapsulating them in micro- or even nanomaterials.
Finally, it is important to reiterate that the study and understanding of the fish skin mucus interactome using omic techniques provides new, unprecedented opportunities for antimicrobial drug discovery. Multiomics may also allow for the discovery of clinically important metabolites, interactions between components, and the mechanisms by which components exert their antimicrobial activity.