Exogenous antioxidants are those which our bodies cannot produce themselves, including vitamins A (and the related family of carotenoid molecules), C (ascorbate), and E
[25]. Vitamin A is structurally related to β-carotene (a pro-vitamin A compound)
[26], and is composed of two subgroups, retinol (Vitamin A
1) and dehydroretinol (Vitamin A
2). These molecules differ in their antioxidant mechanisms. Vitamin A, which refers to the larger family of vitamins, can combine with peroxyl radicals, acting as a chain-breaking antioxidant before the peroxyl radicals can interact with lipids and generate hydroperoxides, thus preventing cellular damage
[27]. Carotenoids can scavenge singlet oxygen and peroxyl radicals, both of which are highly reactive and unstable
[28]. Additionally, carotenoids may exert indirect antioxidant activity by upregulating SOD and catalase
[29]. Vitamin C is a common exogenous supplement that can scavenge free radicals and has a well-established protective role in carcinogenesis
[30]. Vitamin C is maintained in its reduced form by interacting with glutathione, allowing it to reduce and neutralize ROS
[31][32]. Importantly, vitamin C is capable of regenerating vitamin E in lipid membranes by using reducing equivalents and glutathione. Vitamin E collectively describes a group of related tocophenols and tocopherols
[33]. Of these, α-tocopherol has been highly studied due to its high bioavailability
[34]. This lipid-soluble antioxidant protects lipid membrane oxidation by reacting with lipid radicals that are produced in the lipid peroxidation chain reaction
[35]. This reaction removes the free radicals, preventing the peroxidation reaction from continuing and damaging cell membranes. During this reaction, oxidized α-tocopherol is generated, which interacts with ascorbates who reduce the oxidized α-tocopherol and recycle it back to its antioxidant form
[36]. Additionally, retinol may also interact with tocopheroxyl radicals and regenerate α-tocopherol
[27].
3. Vitamin A
3.1. Source and Forms
Strictly speaking, vitamin A is all-
trans-retinol
[37]. However, vitamin A, as used in this entry, refers to two general groups of compounds with physiologic vitamin A activity: vitamin A precursor carotenoids and non-carotenoid vitamin A precursors and metabolites
[38]. Examples of vitamin A precursor carotenoids include alpha and beta carotene. Non-carotenoid vitamin A precursors and metabolites include retinol esters (inactive storage form), retinal (used for vision), and retinoic acid (potent transcription factor)
[37][39]. Diet is the main source of vitamin A, which includes preformed vitamin A and provitamin A. Preformed vitamin A, as the name suggests, has already been converted by organisms lower in the food chain. Therefore, animal-derived foods are a source of preformed vitamin A (retinol, retinal, retinoic acid, and retinyl esters). Provitamin A consists of plant-derived carotenoids such as beta-carotene alpha-carotene, and beta-cryptoxanthin.
3.2. Antioxidant Activity of Vitamin A
Retinol inhibits peroxidation of liposomes and fatty acid esters in vitro, making it an effective peroxyl radical scavenger
[39]. Compared to tocopherol, retinol’s scavenging ability is even greater, but only when radical species originate from the lipid bilayer and not from the aqueous environment
[39]. Like retinols, carotenoids can also scavenge peroxyl radicals, and they can quench singlet oxygen, a partially reduced oxygen that is highly reactive and unstable. The antioxidant potencies of preformed vitamin A versus carotenoids have been compared via in vitro studies of liposomal systems. Carotenoids with at least 11 conjugated double bonds (beta-carotene, lutein, lycopene, cryptoxanthin, and zeaxanthin) are five times more effective than retinoids such as retinol, retinol palmitate, and retinoid acid in resisting oxidation
[39]. Alpha and beta carotene, lycopene, lutein, and cryptoxanthin constitute the majority of carotenoids present in human plasma. Despite the fact that lutein and lycopene have little to no provitamin A activity, they demonstrate significant antioxidant effects and may be even more potent antioxidants than provitamin A carotenoids
[39]. In other words, it is not necessarily the ability to form vitamin A that gives some carotenoids greater antioxidant potential than others, but rather the intrinsic properties of carotenoids themselves. Interestingly, carotenoid antioxidant activity is greatest at physiologic oxygen tension and becomes less protective in a concentration dependent manner as oxygen tension increases
[39][40]. Therefore, depending on physiological conditions, antioxidant carotenoids can become pro-oxidant
[40]. One possible theory that emerges from this observation is that carotenoids may function as both chemopreventive and chemotherapeutic agents, depending on the cellular environment (
Figure 2).
Figure 2. Non-carotenoid vitamin A precursors and metabolites include retinol, retinal, retinyl esters, and retinoic acid. Specifically, all-trans-retinoic acid demonstrates indirect antioxidant activity by increasing expression of gene products that enhance cellular response to oxidative stress. Carotenoids include both non-vitamin A and provitamin A precursors. Carotenoids, regardless of their vitamin A activity, exhibit direct anti-oxidizing abilities via radical scavenging and singlet oxygen quenching. Furthermore, carotenoids are dynamic agents that can produce either an anti-oxidative or pro-oxidative response, depending on the partial pressure of oxygen in the cellular environment. Malignant cells maintain high intracellular ROS levels, creating high oxygen tension. Under this condition, carotenoids exert a pro-oxidative response by increasing ROS production to generate oxidative stress that will kill cancer cells. This suggests that carotenoids have potential chemotherapeutic effects. In normal, non-neoplastic cells, carotenoids balance the production and elimination of ROS. This suggests that carotenoids may have a role in chemoprevention.
4. Vitamin C
4.1. Antioxidant Effects of Vitamin C
Vitamin C, otherwise known as ascorbic acid, is a hydrophilic vitamin which has hydroxyl groups at a double bond in a lactone ring. This allows the vitamin to be a donor of protons and electrons, which is critical in its ability to reduce ROS, including superoxide anions, hydroxyl radicals, and singlet oxygen (
Figure 3)
[41]. Additionally, vitamin C may prevent cancer by modulating different biological processes. Vitamin C is a critical cofactor for many groups of hydroxylases that are involved in regulating the transcription factor hypoxia-inducible factor 1 (HIF1)
[41]. Elevated HIF activity can foster the stem cell phenotype, making the cancer more lethal due to the tumor cell’s ability to rapidly divide and promote poor blood vessel development. In order to control HIF and prevent tumor development, HIF hydroxylases must tag the protein for degradation. Vitamin C functions as a cofactor for the HIF hydroxylases; therefore, when cells are deficient in vitamin C acid, HIF hydroxylase activity decreases and HIF transcription activity is increased
[41]. When HIF levels are high there is increased tumor growth and development, but with the opposing hydroxylases present, HIF can be managed to prevent tumorigenesis
[41]. Thus, vitamin C is critical for these hydroxylases to function, supporting its possible role in cancer prevention. This has led to the growing research evaluating the addition of vitamin C acid to cancer cells to decrease proliferation
[41]. While it is possible that the anti-cancer effect of vitamin C may be attributed to its role in modulating HIF function, there may be multiple pathways by which this effect occurs.
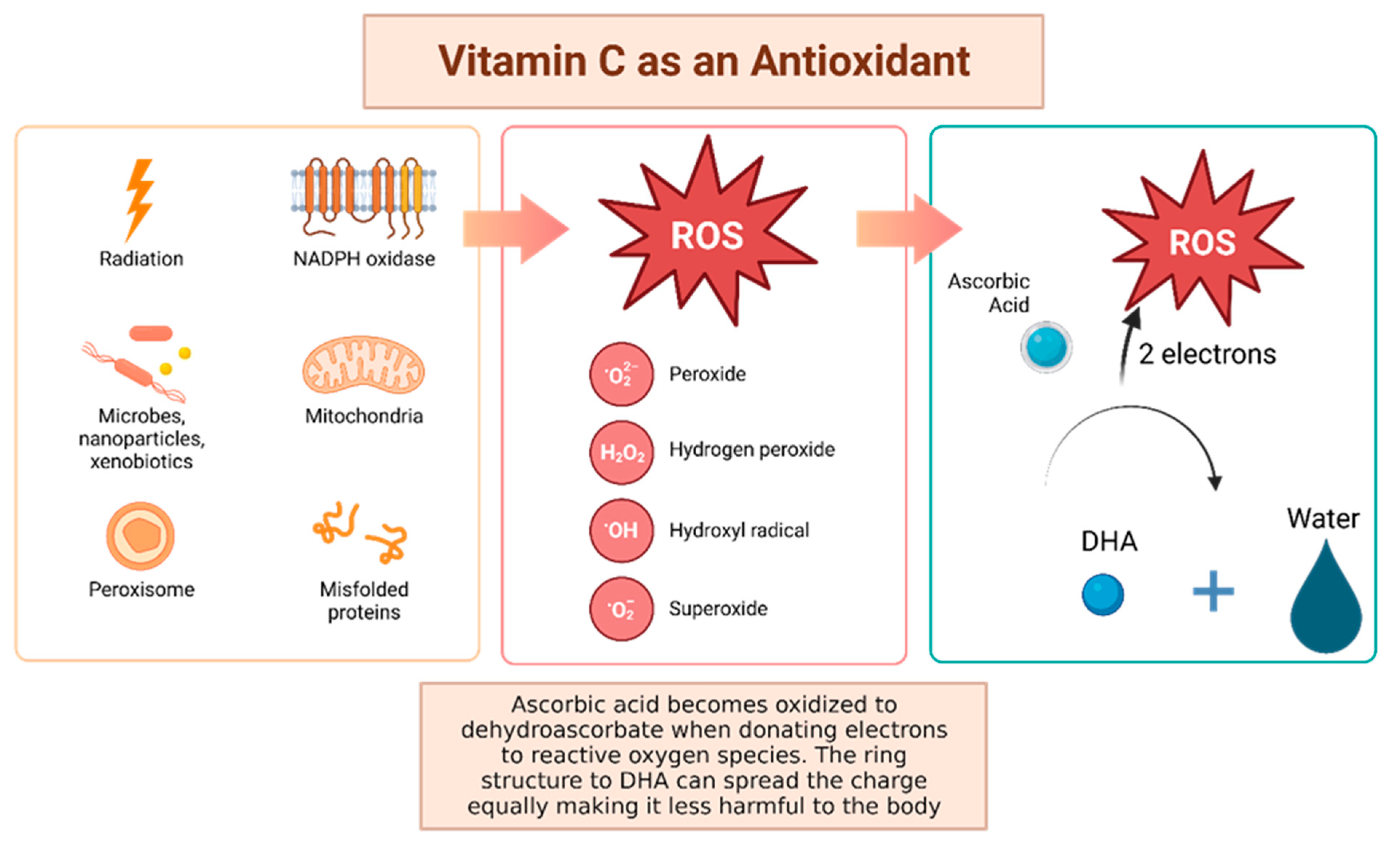
Figure 3. Vitamin C has been shown to have antioxidant properties, allowing it to reduce free radicals that may cause harmful damage to DNA. Reactive oxygen species (ROS) may be made by peroxisomes, radiation, the mitochondria, and more biological processes which result in ROS. Vitamin C acid, when ingested, contains electrons that it can give to reactive oxygen species. These will be reduced to water, and therefore will not be harmful to the body
[41]. The oxidized version of vitamin C, or dehydroascorbate, has the ability he ability to even out the positive charge with its ring structure ensuring that it, itself, is not going to damage cells
[42].
4.2. Vitamin C Has Pro-Oxidant and Gene Regulator Properties
While there is a growing body of evidence demonstrating that vitamin C acts as an antioxidant, it also may contain pro-oxidant functions that lead to cellular damage in vitro. The pro-oxidant features of vitamin C are emphasized when it interacts with metals, such as iron and copper. Here, vitamin C will act as a reducing agent and then form oxygen free radicals
[42]. Interestingly, one mechanism by which vitamin C reduces tumorigenesis may be related to these pro-oxidant capacities. Chen and colleagues evaluated whether pharmacologic doses of vitamin C would reduce tumor growth in mice with aggressive glioblastoma, pancreatic, and ovarian tumor xenografts
[43]. They discovered that vitamin C supplementation led to an increase in vitamin C radical and hydrogen peroxide formation and a decrease in tumor size across all tumor types by 41–53%. This occurred in the interstitial fluid of tumors and not in the blood, suggesting a targeted effect with potentially minimal side effects. Another study evaluated the cytotoxicity of ascorbate, with similar results. Ascorbate was shown to induce apoptosis due to the extracellular generation of hydrogen peroxide
[44]. Given the targeted impact of ascorbate on cancer cells, there is some rationale that this pro-apoptotic effect may occur in newly initiated cancer cells, preventing their proliferation and tumorigenesis.
Additionally, vitamin C may indirectly decrease tumorigenesis via its actions as a cofactor for enzymatic reactions. Peng and colleagues evaluated the role of vitamin C in the transition of 5-hydroxymethlcytosine (5hmC) to 5-methylcytosine (5mC), a methylated form of the DNA base cytosine
[45]. Loss of 5hmC, which corresponds with increasing DNA methylation, is considered to be an important marker of tumorigenesis
[46]. Vitamin C acts as a cofactor for Fe-2-oxoglutarate dioxygenases, which include ten-eleven translocation (TET) enzymes
[47]. TETs reduce DNA methylation by converting 5mC back to 5hMC. Their results demonstrated that vitamin C can increase the content of 5hMC of bladder cancer both in vitro and in vivo, decreasing the malignant phenotype and thus cancer risk
[48]. Additionally, ascorbate has been shown to accumulate intracellularly and promote TET activity in hematopoietic stem cells, decreasing leukemogenesis
[49]. Similar results have been demonstrated in melanoma cells
[50]. Further, vitamin C may inhibit tumorigenesis via mitochondrial dysregulation
[51]. In pancreatic adenocarcinoma cell lines, vitamin C supplementation resulted in decreased cell growth via the inhibition of glucose metabolism without altering the levels of ROS
[51]. The mechanism by which this occurs is largely unknown but is believed to be related to mitochondrial dysregulation because the addition of pyruvate to the medium rescued cancer cells from death. This suggests that vitamin C supplementation may decrease pyruvate concentrations, suppressing cellular respiration.
5. Vitamin E
5.1. Vitamin E Background and Antioxidant Properties
Vitamin E refers to a group of fat-soluble antioxidants known as tocopherols and tocotrienols. These compounds naturally occur in plants, which produce four different homologues (α-, β-, γ-, and δ-) of each depending on the placement of methyl groups on their chromanol ring. Dietarily, these nutrients are abundant in nuts, seeds, oils, and also appear in various other foods in Western diets as a supplemental additive—most commonly appearing as γ-tocopherol. The homologue α-tocopherol is found most predominantly in human tissue and appears to be preferentially absorbed and metabolized. Despite this, all forms of vitamin E share the same antioxidant mechanism, which acts by scavenging for free lipid peroxyl radicals that are biproducts of the lipid peroxidation chain reaction—particularly protecting the lipid membranes of the cell, where vitamin E is often found
[52][53][54][55].
5.2. Vitamin E Induces Anti-Inflammatory Effects
Beyond antioxidant benefits, three variants of vitamin E—γ-tocopherol, δ-tocopherol, and γ-tocotrienol—have been found to have robust anti-inflammatory effects. Specifically, these forms of vitamin E were found to inhibit prostaglandin E2 (PGE
2) and leukotriene B4 (LTB
4) in epithelial cells, macrophages, and in neutrophils without directly inhibiting the enzymatic function of COX-2 and 5-LOX
[53]. On the other hand, the 13′-carboxychromanol metabolite of vitamin E forms appear to inhibit 5-LOX, and the cyclooxygenase activity of COX-1 and COX-2, directly
[53][56][57][58]. Additionally, γ-tocotrienol has been found to be a strong inhibitor of NF-κB activation within various cancer cell lines and lipopolysaccharide (LPS) activated macrophages. In these LPS-stimulated macrophages, the effect of limiting NF-κB activity via γ-tocotrienol decreases IL-6 and granulocyte-colony stimulating factor (G-CSF) production, hindering inflammation
[53][59]. γ-tocotrienol has also been shown to inhibit JAK-STAT3 signaling in cancer cells by activating protein-tyrosine phosphatase SHP-1
[53][60]. Lastly, γ-tocotrienol can limit JAK-STAT6 signaling by blocking the phosphorylation of STAT6 and the ability of STAT6 to bind to DNA
[53]. These factors have made vitamin E variants enticing as both preventative agents and as potential adjuncts to various cancer treatments.
6. Conclusions
Oxidative stress has been implicated in carcinogenesis through a number of mechanisms, including lipid peroxidation, DNA damage, and its impact on both tumor suppressor genes and oncogenes. Thus, there is rationale that supplementation with antioxidants, which counter oxidative stress, may lead to a decrease in cancer risk. However, the current body of in vitro studies paint an inconsistent picture. A number of studies demonstrate evidence of this theory, but other studies fail to show any decrease in cancer formation when cell lines are treated with antioxidant supplements. Similar results were found for observational studies evaluating a link between antioxidant supplementation and cancer risk. Concerningly, some epidemiological studies even showed an increase in cancer risk in patients who used antioxidant supplements. However, these studies were limited by their inability to control for confounders or assess the temporal relationship of oxidative stress and tumor initiation. Large prospective studies following biomarkers of oxidative stress over time and investigating cancer development may address this gap in the literature. Additionally, studies examining the dysplastic transformation of different malignancies are necessary to provide more information regarding changes in oxidative biomarker levels as cancerous lesions progress. The reasoning for the lack of efficacy surrounding antioxidant supplementation as a cancer prophylactic agent is not fully elucidated but may be due to complex and incompletely characterized interactions occurring within the tumor microenvironment or elsewhere within the body. Vitamins may exert a modulatory effect on some components of the tumor microenvironment. For example, vitamin E has been shown to activate antigen-specific immune responses by dendritic cells; vitamin E has also been shown to downregulate suppression of cytotoxic T-cell activation by myeloid derived suppressor cells in leukemia
[61][62].