1. Introduction
Climate change is a complex and multi-faceted issue that affects people and ecosystems in many different ways
[1][2][3]. The issue surrounding climate change is no longer a topic that needs promotion. Although it is a cliche topic these days, it is still a need of the hour. The overwhelming consensus among scientists is that climate change is real, it is happening now, and it is principally caused by human activities
[4][5][6]. The impacts of climate change are already being felt around the world, and they are likely to become more severe and more widespread unless researchers take action to address the problem
[7][8][9]. Its visible key impacts are frequent heatwaves
[10][11], extreme weather events
[12][13], rising sea levels
[14], changes in precipitation patterns
[15], loss in biodiversity with animals rugging to adapt to the rising temperatures
[15], human health, economic impacts, and so on. Despite the urgency of the problem, many people still view climate change as a distant or abstract threat, and they may not be aware of how it is already affecting their lives
[16]. This is partly due to the way that climate change is often discussed in the media and political circles. It is presented as a remote and technical issue that is disconnected from people’s everyday experiences
[17][18]. The truth is that climate change is a very real and urgent threat. The longer researchers wait to address the problem, the more difficult and expensive it will be to solve, and the more severe the impacts are likely to be on people and ecosystems around the world. Fortunately, many solutions to climate change can be acted upon now, considering the necessary knowledge, technology, and resources researchers possess to address the issue. The past decades have seen a plethora of global climate change movements involving individuals, organisations, and governments that work to raise awareness and lay a roadmap for the transition to a more sustainable future. The movement also includes a wide range of actors, from grassroots activists and community groups to scientists, policymakers, and business leaders. There are many different solutions to climate change, and the most effective approach is likely to involve a combination of different strategies. Some of the key solutions to climate change include:
-
Renewable energy: One of the most important solutions to climate change is the transition to renewable energy sources, such as wind, solar, and hydropower. Renewable energy is clean, sustainable, and abundant, and it can help to reduce greenhouse gas emissions and mitigate the impacts of climate change. Some of the renewable energy technologies are solar photovoltaic, wind turbine, hydroelectric power plants, geothermal energy, and tidal energy technologies.
-
Energy efficiency: Another important solution to climate change is improving energy efficiency, which refers to the use of less energy to achieve the same level of service. This can be achieved through a variety of measures, such as better insulation, more efficient appliances, and smarter transportation systems.
-
Carbon capture and storage: Carbon capture and storage (CCS) is a technology that captures carbon dioxide emissions from power plants and other sources and stores them underground, preventing them from entering the atmosphere. CCS can help to reduce greenhouse gas emissions and mitigate the impacts of climate change
[19].
-
Synthetic fuels: Synthetic fuels, also known as e-fuels or artificial fuels, are produced from renewable or non-renewable sources that resemble the characteristics of fossil-derived fuels. They can be used in place of traditional fossil fuels in a variety of applications, such as transportation, heating, and power generation.
-
Carbon pricing: Carbon pricing is a policy that puts a price on carbon dioxide emissions, through taxes or cap-and-trade systems. This provides a financial incentive for businesses and individuals to reduce their emissions and transition to cleaner technologies
[20].
Many of the activities that are essential to modern life, such as transportation and power generation, rely heavily on fossil fuels, which release large amounts of carbon dioxide and other greenhouse gases into the atmosphere. This contributes to global warming and other environmental problems. Synthetic fuels, on the other hand, can be produced using renewable electricity, which reduces greenhouse gas emissions compared to fossil fuels
[21]. Further development of such technologies on a large scale can help reduce the overall carbon footprint and mitigate the impacts of global warming. In addition, synthetic fuels can be easily stored and transported, which makes them a more flexible and reliable source of energy than some other renewable options, such as wind and solar power
[22]. Another reason why synthetic fuels are a need for the future is that they can help to improve energy security
[23]. Many countries are heavily dependent on imported fossil fuels, which makes them vulnerable to supply disruptions and price fluctuations
[24].
2. Classification of Synthetic Fuels
Synthetic fuels are chemically synthesised fuels designed to mimic the chemical and physical properties of fossil fuels
[25][26][27]. They are typically made from renewable or non-fossil feedstocks, such as biomass, water, and carbon dioxide, through chemical processes that convert these feedstocks into fuel. They can be used in place of fossil fuels in a variety of applications, including transportation, heating, and electricity generation
[28][29]. They have the potential to reduce greenhouse gas emissions, as they can be made from carbon-neutral feedstocks, such as biomass or captured carbon dioxide. As a result, the greenhouse gas becomes a raw material from which alternatives for gasoline, diesel, and natural gas may be synthesised using renewable energy
[30]. This means that the carbon dioxide emissions associated with their production and use are offset by the carbon dioxide that is removed from the atmosphere during their production. In contrast, fossil fuels release large amounts of carbon dioxide when they are burned, contributing to climate change. They also offer the potential to provide a more stable and reliable source of energy than electric batteries for transportation
[29]. While electric batteries are a clean and efficient source of energy, they rely on the availability of electricity from the grid, which can be disrupted by outages or natural disasters. Synthetic fuels, on the other hand, can be stored and transported like traditional fossil fuels, making them more resilient in the face of disruptions
[31].
One further key benefit of the combustion engine utilizing synthetic fuels is that the current filling-station network may continue to be used
[32]. The commercial adoption of synthetic fuels is still in its early stages, but the number of companies and organisations that are implementing them is on the rise
[33]. With the degree of public subsidies for renewable energy production reducing and capacities growing increasingly popular, the direct government costs of subsidizing renewable energy are becoming more monitored and understood
[34][35][36]; however, the implicit costs of welcoming higher levels of primary electricity are still largely unpredictable
[37]. A large portion of this is due to the rising costs of balancing generation capacity and consumption in future systems where fuel-based generation will play a smaller role. The capacity to manage future energy systems is difficult to envision without the advantage of fuels of some kind. If fossil fuels are constrained by environmental or other factors, then synthetic fuels appear to have a solid case to take their place. As the demand for renewable and sustainable sources of energy continues to grow, synthetic fuels are likely to play an important role in the global energy mix
[38][39]. Despite this, significant efforts are still required before synthetic fuels may be developed on a commercially large scale since their processing facilities are nevertheless costly and only a few test factories exist
[40][41]. The increased availability of, and hence, lower prices for, renewable energy will also contribute to the widespread usage of these fuels. Furthermore, even if electric cars offer higher efficiency and show the potential to become substantially less expensive in the upcoming years, research into alternative fuels may be important considering their availability and comparable efficiencies with fossil fuels. A comparison of current efficiencies is illustrated in
Figure 1.
The future landscape for synthetic fuels is uncertain, as it will depend on the development and deployment of new technologies and the adoption of policies that support their use. However, synthetic fuels will likely play a larger role in the energy mix in the future as a way to reduce greenhouse gas emissions and improve energy security.
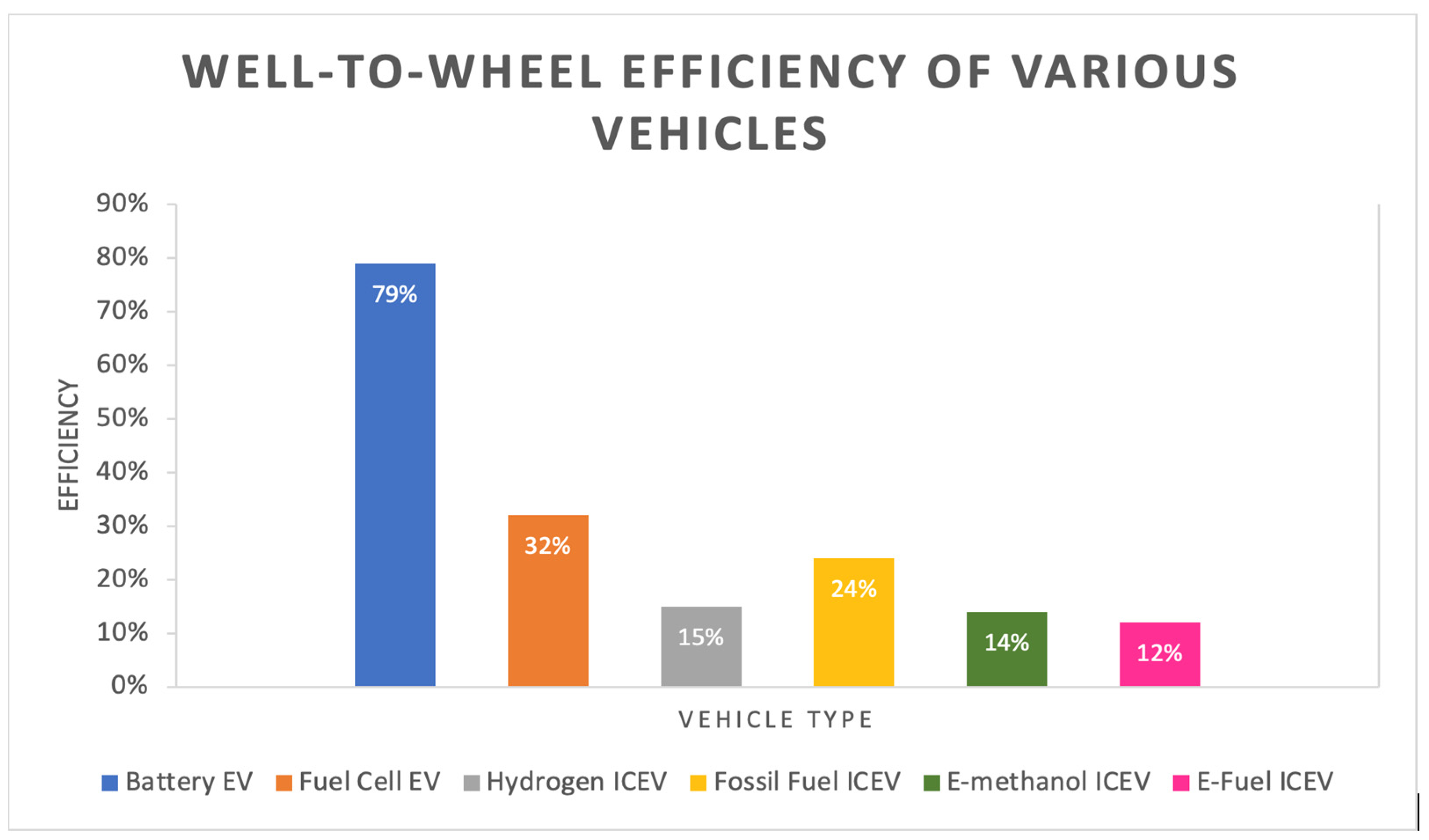
Figure 1. Comparison of well-wheel efficiency between vehicles of various fuels.
The classification of synthetic fuels as broad, mutually exclusive groups is a challenging attempt and is contextual since it depends on various discussed factors such as production process, feedstock, application, sustainability, and chemical composition. For simplicity, this research has adopted the method of classifying synthetic fuels based on their feedstock as it allows the formation of thicker groups of similar fuels. This classification system can aid in a better understanding of the vast number of the major synthetic fuels. The feedstock-based classifications are
-
Biofuels: These are synthetic fuels that are produced from biological materials, such as vegetable oils, animal fats, or waste products. Examples of biofuels include biodiesel, bioethanol, and biogas.
-
Hydrogen fuels: These are synthetic fuels that are produced by reacting hydrogen with other molecules, commonly carbon dioxide. Examples of fuels considered under this category include methanol, DME, and synthetic natural gas.
-
Power-to-liquid (PtL) fuels: These are synthetic fuels that are produced by using electricity from renewable sources to convert water and carbon dioxide into liquid fuel. Examples of PtL fuels include synthetic gasoline, synthetic diesel, and synthetic aviation fuel.
-
Gas-to-liquids (GtL) fuels: These are synthetic fuels that are produced by using natural gas or other gases as feedstocks. Examples of GtL fuels include synthetic diesel, synthetic gasoline, and synthetic aviation fuel.
3. Biofuels
Biofuels are synthetic fuels produced from vegetable oils, animal fats, and other biomass
[42][43]. The feedstock for biofuels is recently decomposed or live plant material, as well as livestock wastes, where feedstock refers to the raw material that is given as input for the production of fuel. The source vegetable oil used for feedstock purposes is extracted from non-edible seeds. For instance, in India, non-edible seeds such as Mahua, Jatropha, Karanja, and Neem are used to extract the oil necessary for the production of biodiesel
[44]. The animal fat feedstock is acquired from slaughterhouses and fish oil from fish processing industries, while other biomass feedstocks include sugarcane bagasse, rapeseed harvest, olive, agricultural residue, and waste cooking oil
[45]. This is in contrast to fossil fuels, which are made from long-dead flora and fauna debris and are non-renewable. Biofuels, on the other hand, are considered to be a renewable source of energy, and their feedstock may be grown again to make biofuels indefinitely. Since they are mostly made from plant-derived materials, carbon dioxide (CO
2) levels do not rise when they are utilised (combusted), which is the idea of carbon neutrality
[46]. At the same time, their similarity with fossil fuels makes them an attractive solution to use as an alternative fuel in places where fossil fuels are currently used; for example, they can be used in internal combustion engines.
Depending on the type of feedstock used, biofuels have been categorised into four under the name of “generations”
[47]. The first-generation biofuels have their feedstock derived purely from food-based sources. Here, food-based refers to plants and animals that are consumed as food by humans. These include starch, cereal crops, vegetable oils, sugarcane, and animal fats from meat. This generation is predominantly made with the help of microorganisms that ferment the feedstock and is later processed to produce the desired biofuel
[48]. The second generation is produced from non-food sources such as wood, straw, and all the other remaining parts of harvested crops after removing their edible parts
[48]. Owing to their high cellulosic content, they are also called cellulosic biofuels. The second generation also includes non-edible animal fats and oils. The third generation is produced by specific oil-producing algae species owing to their otherwise common name of algal biofuels. This generation of biofuel is low-cost yet limited in stock for production; therefore, large-scale production through these means is still under development
[49]. The fourth generation, the final and latest sector, is produced through advanced techniques of biochemistry in sophisticated lab facilities. However, this generation is experimental and would require a time window to be commercially adopted
[50]. The generational classifications are illustrated in
Figure 2.
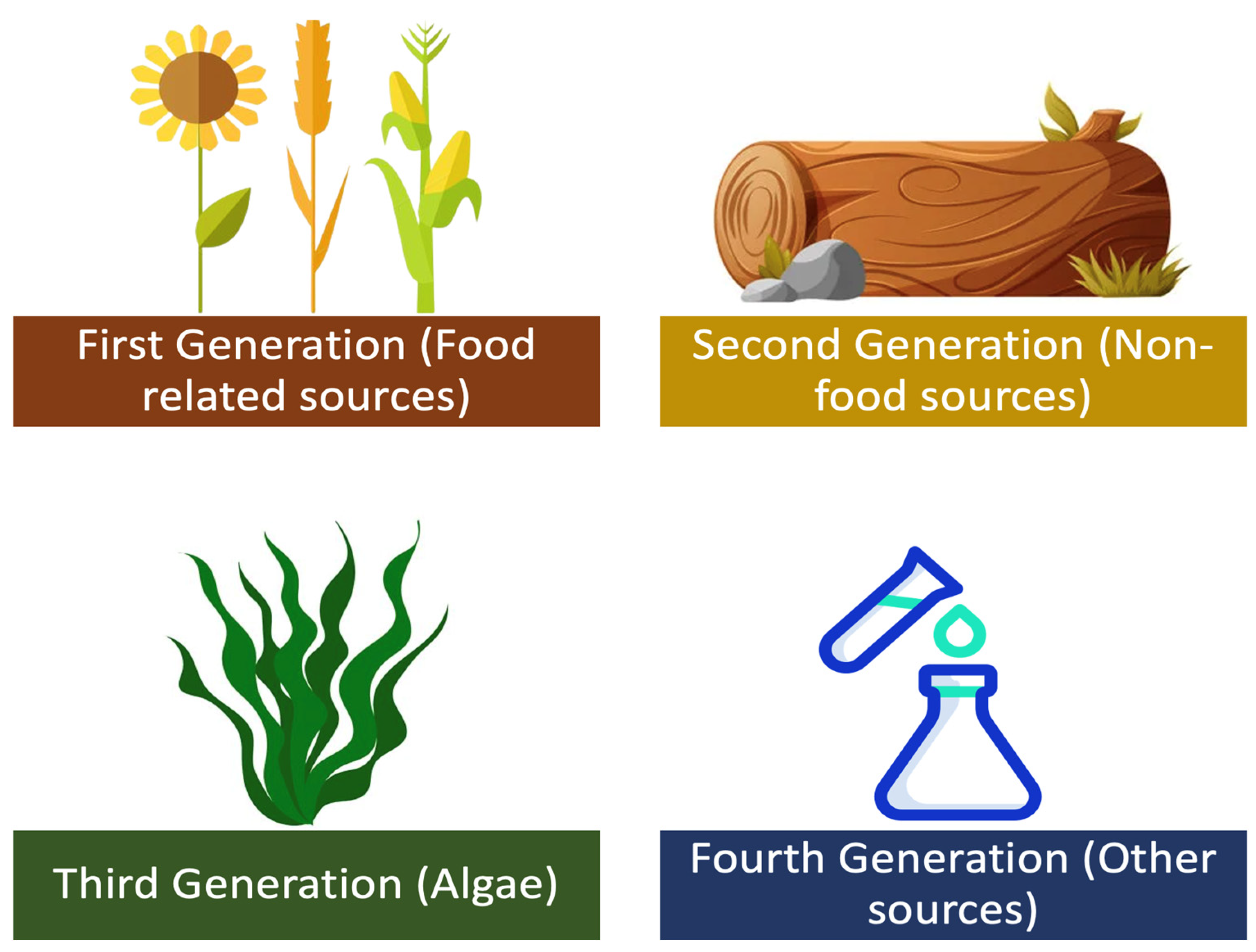
Figure 2. Generations of biofuels.
The advantages of biofuels include biodegradability, non-inflammable nature, low toxicity, safety, high combustion efficiency, abundance, lubricant nature, high cetane number, lower emission, and renewability. Their disadvantages include low calorific value, nitrous oxide emissions, poor shelf life, and their tendency to cause engine wear [51][52]. They can be used in their pure form and can even be blended with fossil fuels owing to their miscibility [53]. The nomenclature for biodiesel blends is denoted as Bxx, where xx represents the percentage of biofuel in the blend. For example, B40 indicates a 40% biofuel and 60% fossil fuel mixture. A blend of up to B20 can be used in existing internal combustion engines without any structural design changes [54].
3.1. Biodiesel
Biodiesel is composed of fatty acid alkyl esters (FAAEs) and is considered a viable low-carbon alternative for fossil-based diesel, particularly in the transportation sector [55][56]. Biodiesel can be produced through four main routes: oil-blends, micro-emulsion, pyrolysis, and transesterification. The transesterification route is the most preferred due to the quality of fuel produced [57]. Transesterification is an eco-friendly chemical process in which the fatty acids in vegetable oil or animal fat are converted into esters [53]. This reaction is typically carried out using a catalyst, such as sodium hydroxide or potassium hydroxide, and an alcohol, such as methanol or ethanol [58][59]. The parameter with the highest impact on the yield is the temperature, followed by reaction time and pressure. The general reaction of transesterification reaction is depicted in Equation (1).
In this reaction, the fatty acids in the vegetable oil or animal fat react with the alcohol to produce biodiesel and glycerol, where glycerol is a by-product of the reaction and is typically removed and used in other applications, such as soap or animal feed [60][61]. The production of biodiesel is a relatively simple and straightforward process, and it can be carried out on a small or large scale, using a variety of different feedstocks. Biodiesel is a versatile fuel that can be used in a wide range of applications, and it offers several advantages over petroleum-based diesel, including improved air quality, reduced greenhouse gas emissions, and improved energy security [62][63][64]. It is important to note that FT-diesel (covered in later sections), produced through biomass feedstock, is also commonly called biodiesel; however, it is not the same as the FAME biodiesel discussed in this section [65]. A significant obstacle to biodiesel’s widespread commercialisation is its high price and poor efficiency [66]. The high cost of feedstock and lack of infrastructure for moving significant amounts of biodiesel from refineries to major population areas further restrict the availability of this fuel.
3.2. Hydrogenated Vegetable Oil (HVO)
HVO is an alternative fuel made from a variety of vegetable oils and fats containing triglycerides and fatty acids. HVO is another name for hydro-processed esters and fatty acids (HEFA) [67]. It is also known as renewable diesel or green diesel. It is generated through hydrogenation and hydrocracking of paraffinic hydrocarbon forms of lipids where its feedstock is composed of vegetable oil, tallow, and animal fat [68]. Rapeseed is found to produce the highest product yield of HVO of all the feedstocks [69]. Although HVO is obtained from similar feedstocks as biodiesel, it is produced through a hydrotreated process, as opposed to biodiesel, which is obtained through transesterification [70]. This difference helps in improving the oxidation stability of HVO. This results in better resilience to bacterial growth in comparison to biodiesel, making it a better solution for longer and standby applications [71]. Its chemical characteristics are comparable to those of fossil diesel, but differ from diesel with lower density and energy content [72]. It also has a high cetane number and is devoid of sulphur, oxygen, and aromatic hydrocarbons [73]. It is now the second largest renewable diesel alternative, and it is blended with fossil diesel combinations, which are now offered at petrol stations [74][75][76]. The production flow diagram of HVO is illustrated in Figure 3. The general reaction for HVO production is depicted in Equation (2).
The production of HVO is a complex and multi-step process, and it requires specialised equipment and expertise [77]. In the process shown in the equation, the fatty acids in the vegetable oil or animal fat are first converted into paraffin, olefins, and naphthene using a series of chemical reactions, which is followed by combining these chemicals to form the HVO fuel [78][79]. The resulting fuel has a number of advantages over biodiesel, including improved stability, improved energy density, and improved handling characteristics. As a result, HVO is increasingly being used as a replacement for petroleum-based diesel in a variety of applications. Although HVO is comparably better than biodiesel and synthetic diesel with respect to cetane number and other fluid properties, it requires a high capital for its production compared to synthetic and biodiesel [80].
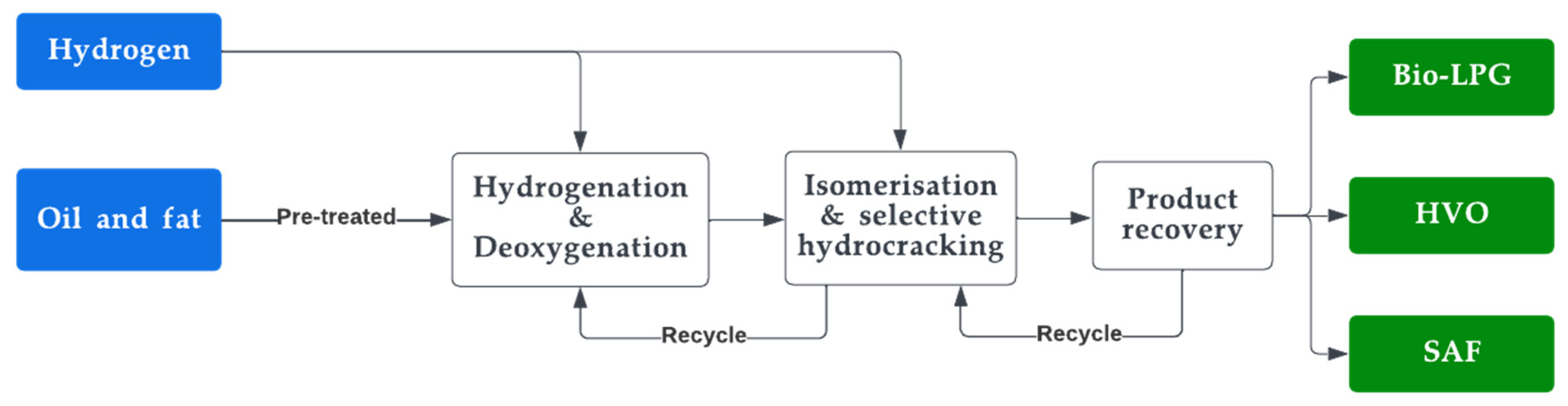
Figure 3. HVO production process.
3.3. Bio-Methanol and Bioethanol
Bioethanol is a type of biofuel that is produced by fermenting biomass, such as corn or sugarcane, with yeast [81]. The resulting liquid can be blended with gasoline to create a fuel that can be used in conventional gasoline engines [82][83]. Mixing bioethanol with unleaded petrol in a ratio of 10% bioethanol and 90% petrol is known as E10, which is commonly offered at filling stations. Another mixture, E85, also exists and contains 85% of ethanol in the mixture. However, this mixture cannot be continuously used on conventional engines as it would eventually result in wear [84][85]. Nevertheless, a specially designed engine for E85 is promising and offers engine operation at lower temperatures [86][87]. Bioethanol has several advantages over gasoline, including reduced greenhouse gas emissions and improved air quality [88]. It is worth noting that, chemically, there is no difference between ethanol and bioethanol. The difference in the name implies that bioethanol is ethanol produced through the biochemical process of fermentation which predominantly uses biological matter as feedstock. The production of bioethanol involves several steps, including harvesting the biomass, grinding it into a fine powder, and mixing it with water and yeast to create a mash. The mash is then fermented, typically using a type of yeast called Saccharomyces cerevisiae [89], which converts the sugars in the biomass into alcohol. The alcohol is then separated from the mash and purified to produce bioethanol. There are various terms coined for the aforementioned processes, namely: saccharification, fermentation, distillation, and ethanol dehydration. The feedstock classification of bio-methanol is the same as that of the general biofuel feedstock classification shown in Figure 2. Currently, most of the biomass produced today is of the first generation, where the most used first-generation feedstocks to produce methanol and ethanol are sugarcane extract and starch [90][91]. Figure 4 illustrates the production of bioethanol and Equation (3) depicts the general reaction of bioethanol production.
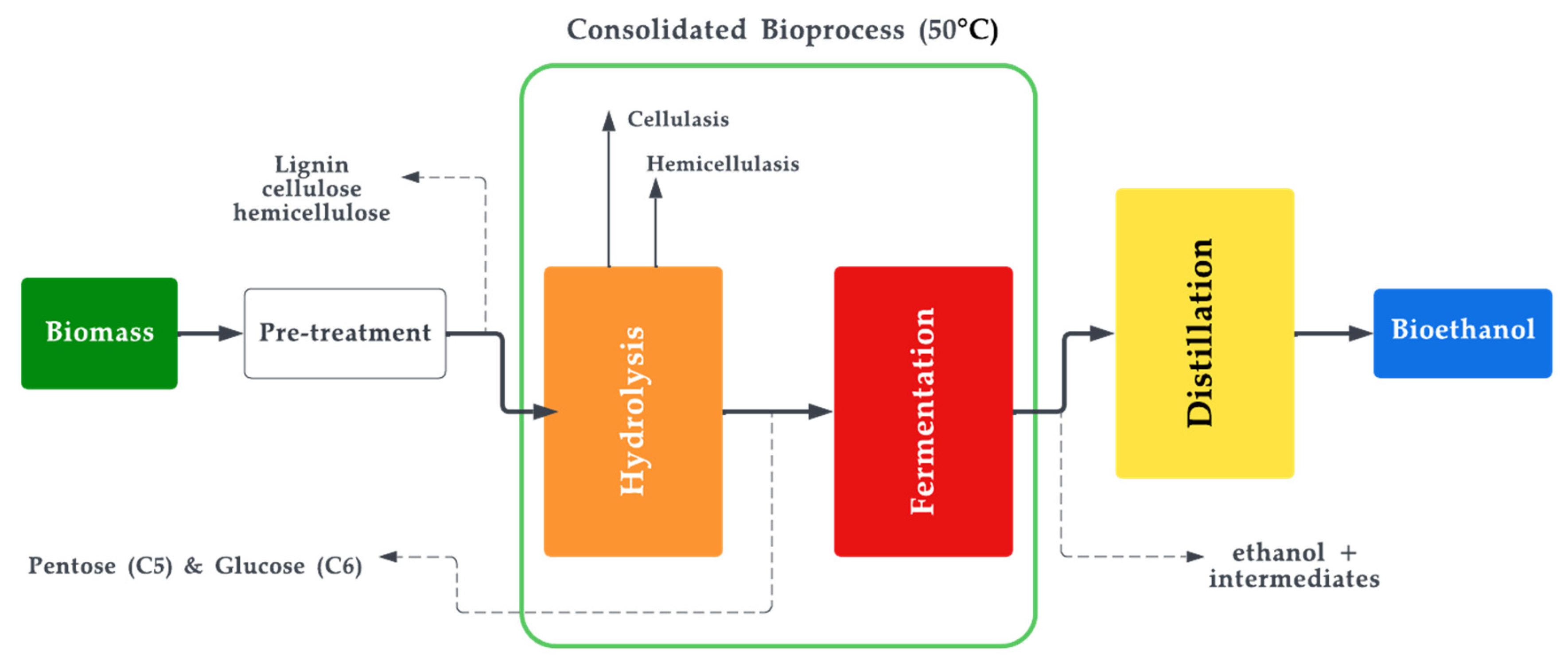
Figure 4. Bioethanol production process.
Production of bio-methanol is similar to that of bioethanol. It is produced through the fermentation of biomass, such as wood or agricultural waste, using microorganisms that are capable of producing methanol [92]. The process is similar to the production of bioethanol, with the main difference being the type of microorganism. The yield of methanol/ethanol is variable and is determined by various factors. Some of the factors include carbon source concentration, fermentation time, temperature, and pH [90]. The general reaction for bio-methanol production through this method is described in Equation (4). It is worth noting that methanol is also produced through the catalytic conversion of syngas commonly around the world [93], which is a mixture of carbon monoxide and hydrogen that is produced from the gasification of biomass. Since the majority of the methods for producing methanol/ethanol include utilizing biomass, solid waste, and carbon capture technologies, the production of methanol and ethanol is sustainable. The reaction for the production of bio-methanol from syngas involves the reverse water-gas shift reaction, depicted in Equation (5), followed by reacting CO with H2, as depicted in Equation (6).
Methanol and ethanol have their own pros and cons: a comparison of some aspects are
4. Hydrogen Fuels
Hydrogen energy is a promising source of clean, renewable energy and has the potential to play a major role in the future of energy production. Hydrogen is the most abundant element in the universe, and when it is burned, it produces only water and heat as by-products. This makes it a clean-burning fuel that does not produce any harmful greenhouse gases or other pollutants. The three major ways of producing hydrogen are steam reforming, electrolysis, and through biological processes. Steam reforming involves reacting methane with steam to produce hydrogen gas, while electrolysis involves splitting water into hydrogen and oxygen using electricity. Biological processes, on the other hand, involve using microorganisms to produce hydrogen from organic matter. One of the major challenges in the production of hydrogen is its cost
[99]. Hydrogen is primarily produced from fossil fuels, which makes it carbon-intensive. However, as renewable energy sources become more widely available and cost-effective, it is likely that hydrogen production will become more sustainable and affordable. Another major challenge is the lack of infrastructure for the distribution and storage of hydrogen
[100]. Hydrogen is transported and stored in a gaseous form, which is unsafe and requires expensive specialised equipment. New technologies are being developed to address these challenges and make hydrogen energy more practical and widespread.
One of the major uses of hydrogen is in the production of synthetic hydrogen fuels. These fuels are made by combining hydrogen with other elements, such as carbon, oxygen, or nitrogen
[101]. For example, methanol is made by combining hydrogen and carbon dioxide
[102], while DME is made by dehydrating methanol
[103]. DME and methanol are considered to be better fuels than hydrogen for several reasons. To mention a few, they are less flammable making them safer and they are renewable as they can be produced from a variety of renewable resources, such as biomass and waste materials
[104]. Hydrogen, on the other hand, is also capable of being produced using renewable sources but is currently produced from fossil fuels when viewed on a large scale. The CO
2 required is captured from the air or from industrial emissions through a variety of methods, namely chemical absorption, adsorption, and membrane separation
[105][106][107][108][109]. In chemical absorption, it is captured by reacting it with a chemical solution that binds to it, forming a compound from which it can be easily separated and stored
[110]. In adsorption, it is captured by passing it through a material (unlike chemical solution in absorption) that binds to it, allowing it to be easily separated and stored
[111]. In the membrane separation method, it is captured by passing it through a membrane that only allows CO
2 molecules to pass through, allowing it to be easily separated and stored
[112]. This entire process of capturing and utilizing CO
2 is called Carbon Capture and Utilisation (CCU). The hydrogen produced through this method is coined the ‘Blue Hydrogen’
[113][114][115][116].
Figure 5 shows the entire cycle of capturing CO
2, producing H
2 and synthetic fuels on an industrial scale.
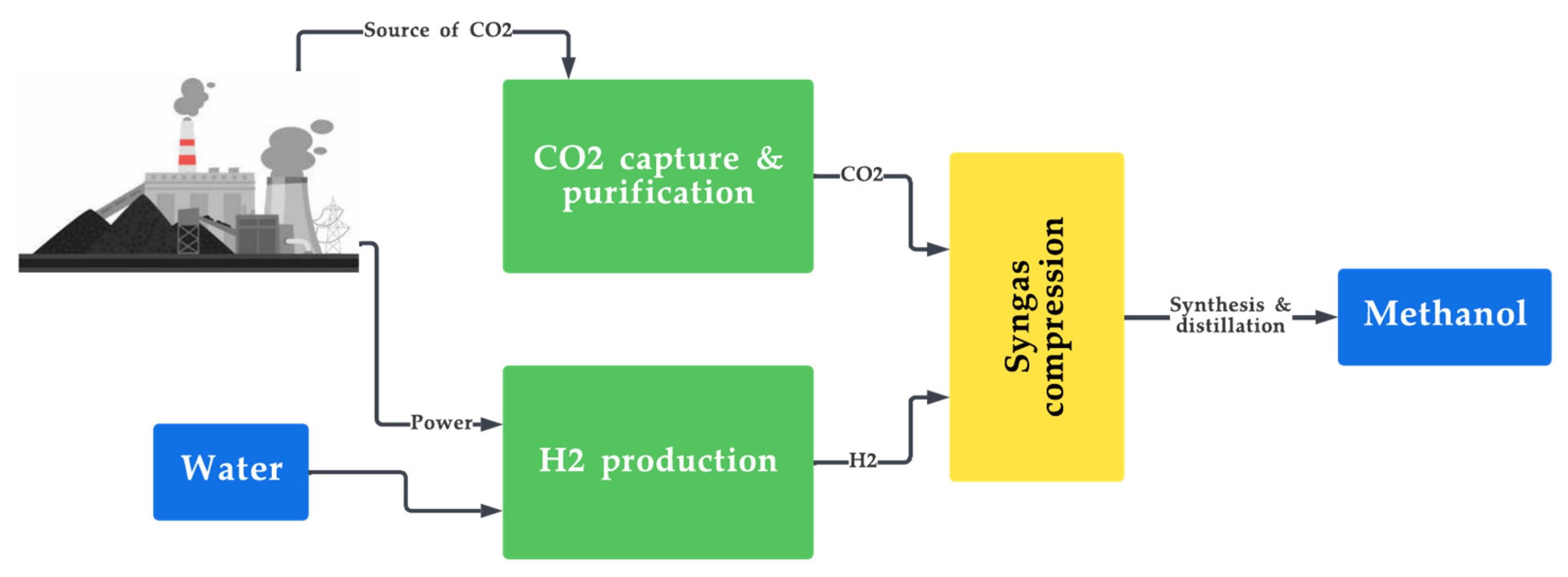
Figure 5. Production of synthetic fuels from CO2 capture and H2 production.
Hydrogen-based synthetic fuels come with their own set of pros and cons. Some of the advantages include the abundant and renewable nature of its feedstock (H2 and CO2) and its ability to be produced locally, eliminating the need for long-distance transportation and thus improving energy security [117][118][119]. Its major disadvantage is its high cost of production as it requires complex and exclusive infrastructure [120][121].
4.1. Hydrogen
Hydrogen energy is a form of clean, renewable energy that can be used for a wide range of applications. It is produced through the process of electrolysis, in which electricity is used to split water into hydrogen and oxygen. There are three main types of hydrogen energy: grey hydrogen, blue hydrogen, and green hydrogen. Grey hydrogen is produced from fossil fuels, such as natural gas, and is the most common type of hydrogen energy. It has high energy density but its production releases carbon dioxide into the atmosphere, contributing to climate change [122]. Green hydrogen, on the other hand, is produced from renewable energy sources such as solar or wind power and is currently being promoted for large-scale adoption for a variety of applications. All three major colour codes of hydrogen are illustrated in Figure 6. In general, hydrogen energy can be used in a number of ways. To name a few:
-
As a fuel for vehicles: It can be used to power cars, buses, and other vehicles. It has a high energy density and can be used to store and transport energy, making it a potential alternative to gasoline and other fossil fuels.
-
For electricity generation: Hydrogen can be burned in a fuel cell to produce electricity, with water as the only by-product. Fuel cells are highly efficient and can be used to power homes, businesses, and other facilities
[123].
-
For industrial processes: It can be used as a chemical feedstock in various industrial processes, such as the production of chemicals, fertilizers, other synthetic fuels, and steel
[124][125].
-
For heating and cooling: Hydrogen can be used as a fuel for heating and cooling systems, providing an alternative to natural gas and other fossil fuels.
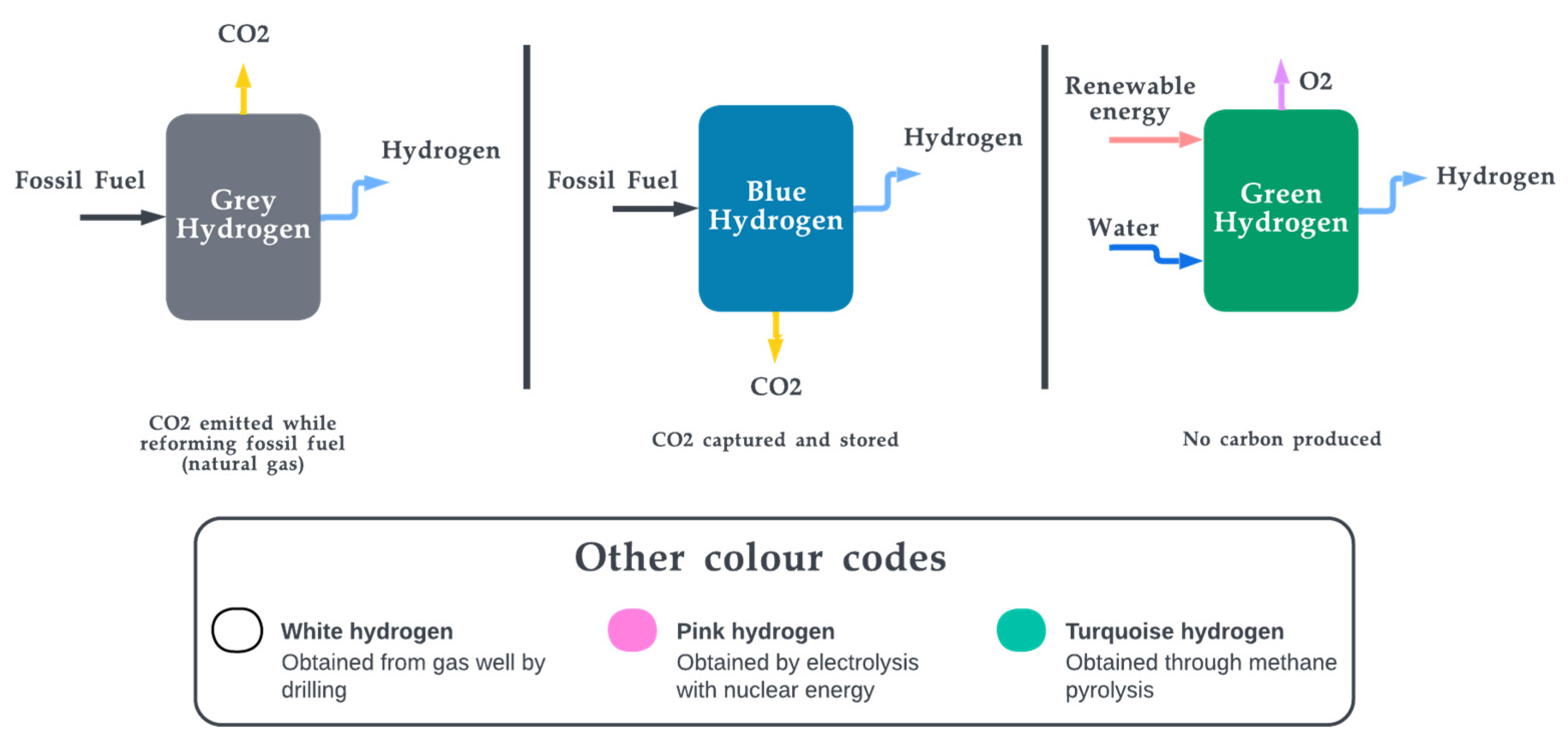
Figure 6. Popular hydrogen colour codes.
Hydrogen is very reactive and the method that produces the purest form of hydrogen is called electrolysis. In this process, electricity is consumed to split water into hydrogen and oxygen. It is one of the most common methods for producing hydrogen energy and can be used to produce both grey and green hydrogen, depending on the source of electricity used. There are several types of electrolysers available, including alkaline electrolysers, proton exchange membrane (PEM) electrolysers, and solid oxide electrolysers
[99]. Alkaline electrolysers use an alkaline solution, such as potassium hydroxide, as the electrolyte
[126]. They are relatively simple and inexpensive to operate, but they are not as efficient as other types of electrolysers
[127]. PEM electrolysers use a proton exchange membrane as the electrolyte. They are highly efficient and can operate at a wide range of temperatures, making them suitable for use in a variety of applications. Solid oxide electrolysers use a solid oxide material as the electrolyte. They can operate at high temperatures and are highly efficient, but they are more expensive to produce and maintain than other types of electrolysers
[128]. This type is used for large power applications. The parameters that have a significant effect on the yield of hydrogen include temperature, electrolyte concentration, and electrolyte flow rate
[129]. The hydrogen produced through electrolysis is typically stored in high-pressure tanks, cryogenic tanks, or metal hydride tanks. The third type is predominantly used these days
[130]. The electrolysis process is illustrated in
Figure 7 and the overall equation for the electrolysis of water is depicted in Equation (7).
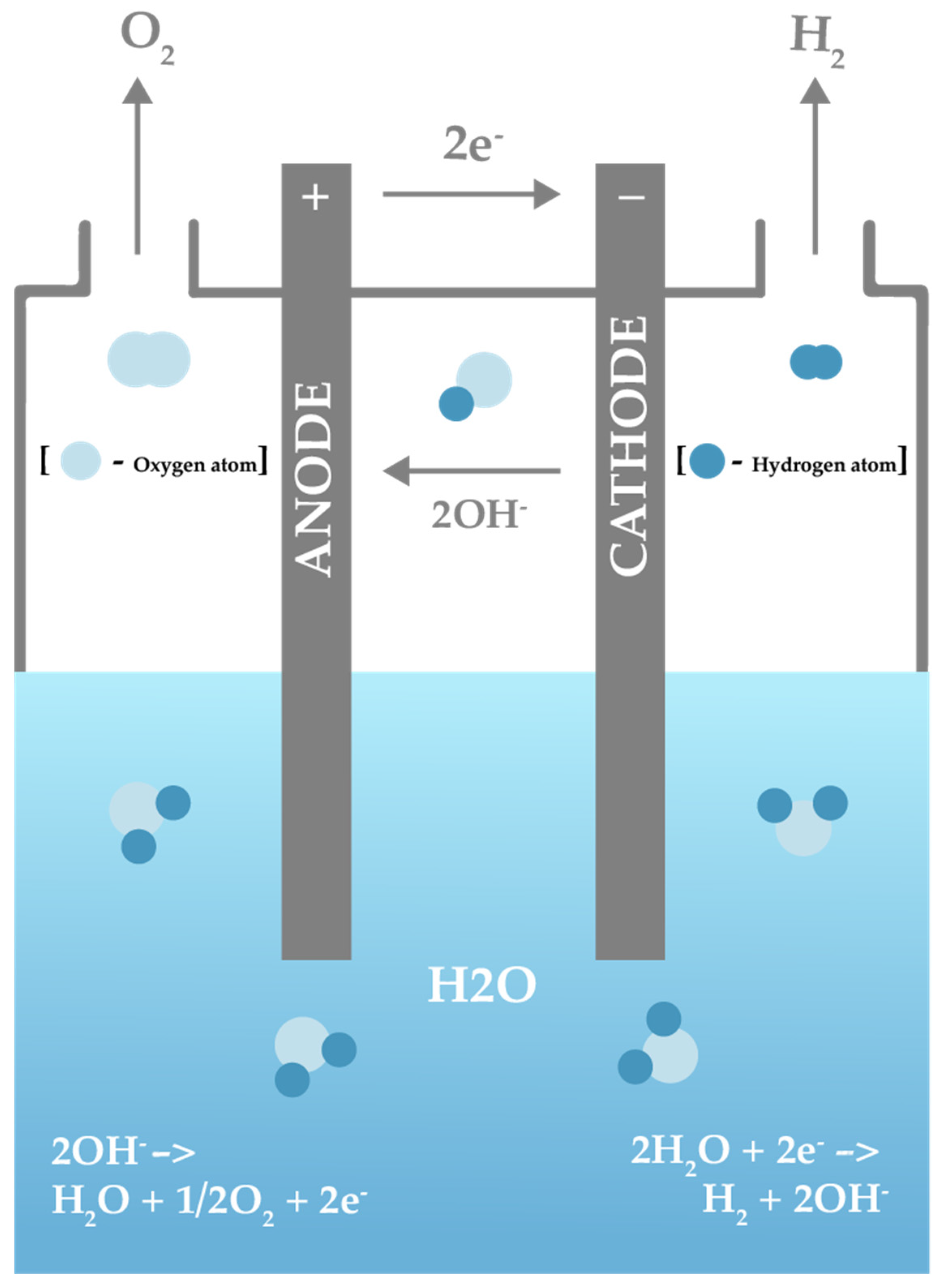
Figure 7. Electrolysis reaction.
Hydrogen energy is being considered to have the potential to play a significant role in the transition to a cleaner and more sustainable energy system on a global scale. As renewable energy sources become more widespread, the production of green hydrogen will increase, helping to reduce the reliance on fossil fuels and decrease greenhouse gas emissions. Currently, worldwide energy organisations are focusing to reduce the costs of producing hydrogen and improving the efficiency of existing systems [131]. An example of one such organisation is IRENA [132]. On the flip side, several academists and professors started highlighting the hydrogen economy to be a scam hosted by fossil fuel industries to delay the current green energy transition [133][134][135][136]. This theory is proposed based on the facts built on the practical efficiencies and costs of incorporating hydrogen systems into the energy transition.
4.2. Syngas
Syngas, also called synthesis gas, is a mixture of carbon monoxide (CO), H2, and CO2 gases that can be used as a fuel or as a feedstock for producing chemicals and other synthetic fuels. Syngas is typically produced through a several-step process with their feedstock being carbon-containing materials, such as coal or biomass [137]. It is produced through the gasification of heavy hydrocarbons and steam reforming or partial oxidation of light hydrocarbons. First, a carbon-containing feedstock is gasified by heating the feedstock in the absence of air but in the presence of partial oxygen, which causes it to break down into its constituent gases. The mixture usually contains H2, CO2, CO, CH4 and nitrogen (N2), where the N2 is inactive and does not participate in any reaction [138]. The preferred composition varies depending on the application, with H2/CO ratios of two or higher for methanol and Fischer–Tropsch synthesis, while a higher ratio is preferred for hydrogen production. DME, higher alcohols, oxo-alcohols, and ethanol are all synthesised from lower H2/CO ratios of around one [139]. When there is a requirement for the ratio to be reduced, the water–gas shift reaction (WGSR) is employed to increase the concentration of H2 and reduce the amount of CO by reforming the mixture with steam and making it more suitable for use as a fuel or for production of other fuels [140]. In contrast, the ratio is increased by converting CO2 content in the mixture into CO through the Reverse-WGSR, also known as catalytic hydrogenation of CO2, whereby the mixture is subjected to high temperature and pressure in the presence of a catalyst, typically a metal such as nickel or cobalt [141][142][143][144]. During the production stages, the composition depends on the reaction conditions and technology employed. The temperature and pressure at which the gasification takes place, as well as the type of feedstock used, can be varied to produce syngas with different compositions and properties. The most important parameters that determine the quality of syngas are the equivalence ratio (ER) and feedstock ash content. The syngas equivalency ratio is a measure of the ratio of the actual fuel–air ratio to the stoichiometric fuel–air ratio necessary for full syngas combustion, where the value of the ratio indicates the quantity of air present to burn the fuel [145][146]. High-quality syngas may be generated at any temperature and pressure combination by adjusting the optimum ER ratio. Figure 8 illustrates the process of producing syngas. The sustainability of syngas production depends on the method and feedstock used to produce it. Syngas produced from fossil fuel sources can be environmentally detrimental. In addition, even when renewable feedstocks are used, the production process requires significant amounts of energy, which often comes from fossil fuels, thus imposing sustainability challenges.
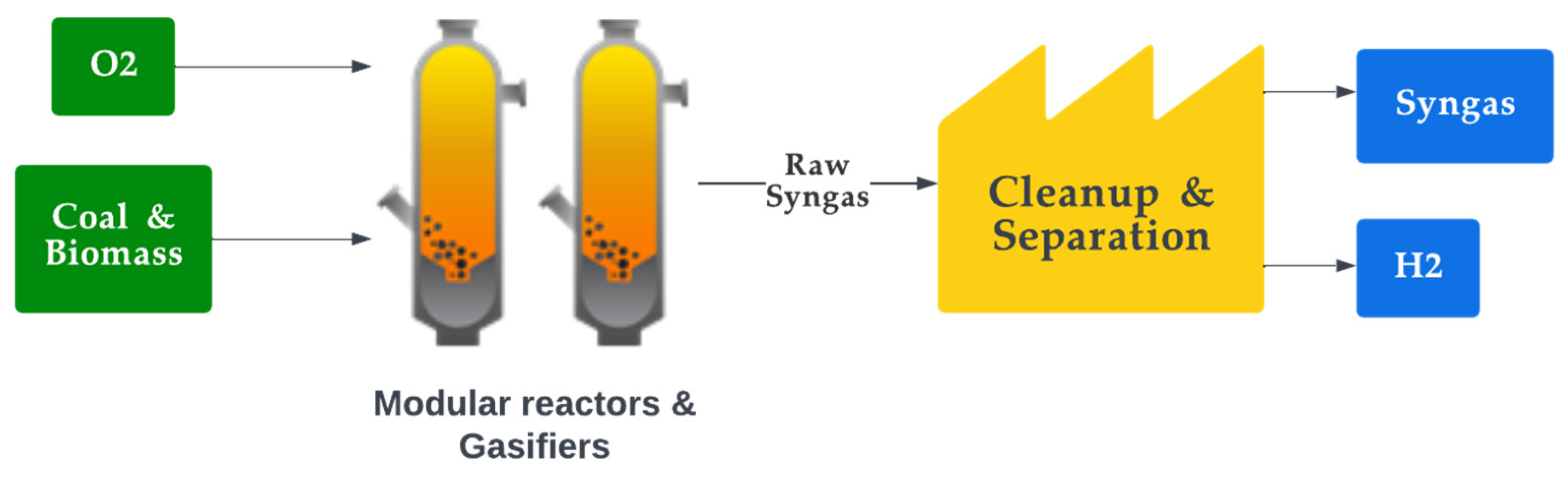
Figure 8. Production of syngas.
Syngas is commonly used for the production of chemicals, such as methanol and synthetic natural gas (SNG), as well as for the production of transportation fuels, such as diesel and jet fuel [147][148]. The technology required for stable production of syngas production from the gasification of biomass continues to be in its infancy, making commercial-scale production a bottleneck [149]. Figure 9 depicts the various uses of the syngas [150].
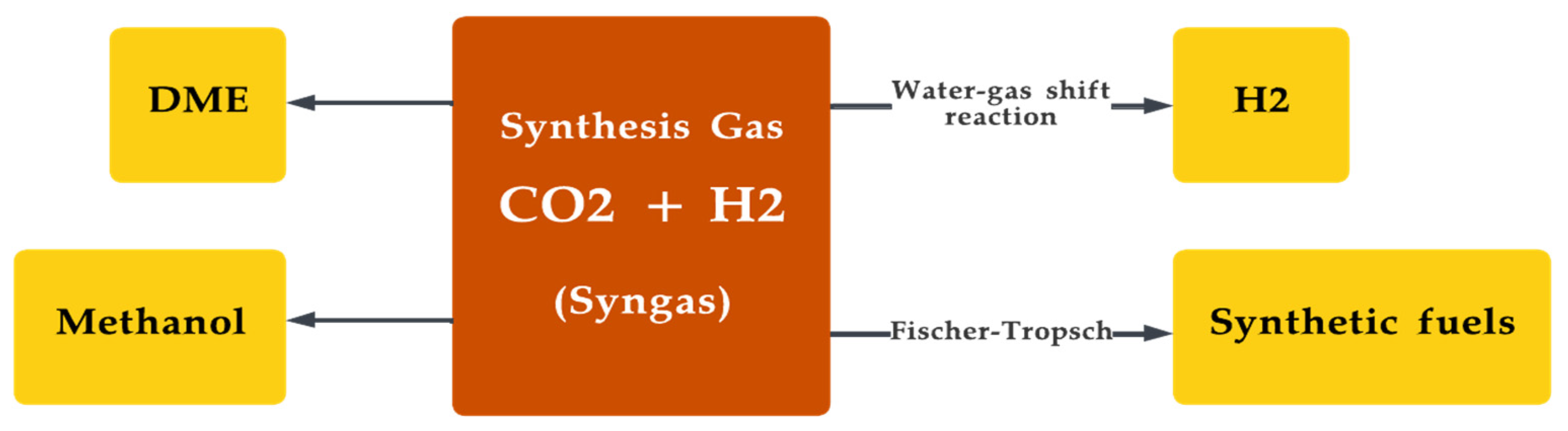
Figure 9. Production of various synthetic fuels from synthesis gas.
4.3. Methanol
Methanol is a flammable liquid that is commonly used as a solvent, a fuel, or a chemical intermediate
[151][152][153]. It can be used in a variety of applications, including as a fuel for vehicles and as a feedstock for the production of other chemicals and materials. When used as a fuel, it produces relatively low levels of carbon monoxide and particulate matter and does not produce sulphur dioxide, which makes it a cleaner burning fuel
[154][155]. One of the main advantages of methanol is that it can be produced from a variety of renewable and waste materials, such as biomass, waste gases, and waste plastics. Some of the sources of waste gases include coal oven gas, landfill gas, gas from palm kernel shell (PKS) solid waste and gas from empty fruit bunch (EFB) solid waste
[156][157][158]. The source of plastic is majorly from the product and plastic packaging composition of municipal solid waste. This makes it a potential alternative to fossil fuels and a way to reduce greenhouse gas emissions and air pollution. There are several methods for producing methanol, the existing methods are:
-
Hydrogenation of carbon monoxide: In this process, carbon monoxide is reacted with water to produce methanol and hydrogen
[159]. On an industrial scale, the CO required for this process is obtained by reforming natural gas
[93]. This method will be discussed in detail in this section.
-
The fermentation of biomass: In this process, biomass, such as wood or agricultural waste, is fermented to produce methanol. It basically converts methane produced from biomaterial into methanol. This type is also known as bio-methanol and was discussed in detail in the previous sections. This method is typically used on a small scale and it is often used to produce methanol from waste materials or other low-value feedstocks. Figure 10 depicts the feedstocks for all types of methanol.
-
Direct methane to methanol conversion: The industrial process of methane to methanol transformation is carried out through the conventional (indirect) route, which is expensive, owing to its high energy requirements. Direct conversion routes for methane to methanol such as plasma, photocatalytic, supercritical water, and biological routes have been developed to achieve cost-effectiveness and feasibility
[93]. However, these technologies are in their early stages and have a time window of 5 to 20 years for industrial feasibility.
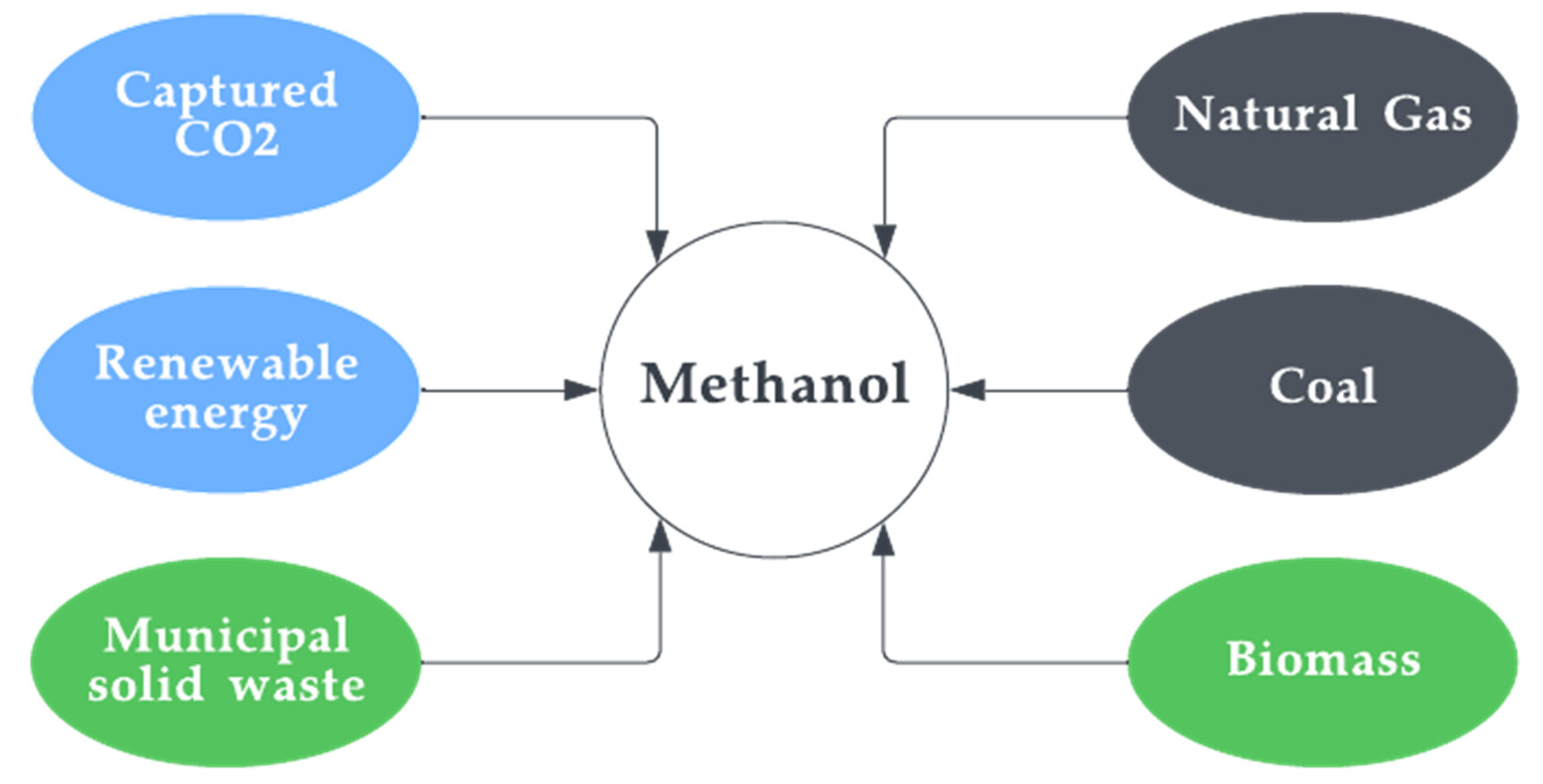
Figure 10. Feedstocks of methanol.
Conventionally, methanol is produced on a large scale from natural gas. This method is considered to be indirect since the natural gas (methane) is first reformed to obtain CO, followed by its hydrogenation in the presence of a catalyst, such as zinc oxide or copper chromite, to produce methanol. The process is typically carried out at high temperatures and pressures, which helps to increase the reaction rate and the yield of methanol. The reaction is exothermic, which means it releases heat, and the heat is typically removed through cooling systems to prevent the reaction from getting out of control. The main advantage of this method for producing methanol is that it can be carried out on a small scale and it can be used to produce methanol from waste gases or other carbon-rich feedstocks. This makes it a potentially economical and sustainable method for producing methanol and a way to reduce greenhouse gas emissions and air pollution. However, there are also some disadvantages to the hydration of carbon monoxide as a method for producing methanol. The process requires specialised equipment and a high-quality catalyst, both of which can add to expenses. Figure 11 illustrates the above-mentioned process pictorially.
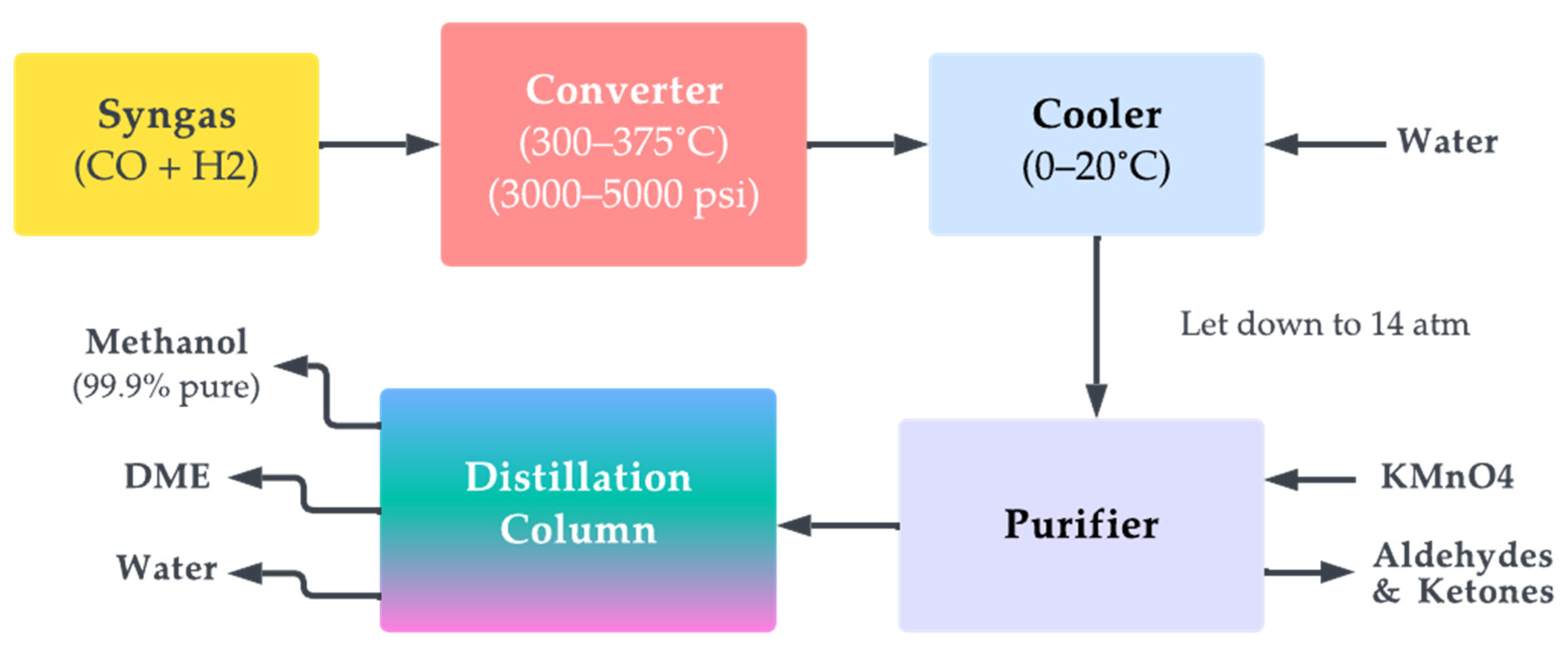
Figure 11. Methanol synthesis process.
4.4. Dimethyl Ethers (DME)
DME is a synthetic alternative to diesel that is intended for use in specifically engineered compression-ignition diesel engines [160]. It has physical characteristics comparable to liquefied petroleum gases and it burns with a visible blue flame and does not generate peroxides in its pure form or in the aerosol formulation. It also produces significantly lower levels of CO2, NOx, SOx, and particulate matter, giving it a significantly lower emissions profile compared to diesel and other fossil fuels [161][162]. It is the simplest form of ether, with a chemical formula of CH3OCH3. Its advantages include clean combustion, high-efficiency compression, the ability to be easily reformed to hydrogen at low temperatures, near non-toxic nature, and cost-effective production and transportation compared to other fuels [163]. The conventional production of DME involves the dehydration of methanol. In this process, methanol is heated in the presence of a strong acid catalyst, such as sulfuric acid, to remove the water molecule and produce DME. As discussed in the previous sections, syngas is first produced from biomass, followed by the simultaneous production and dehydration of methanol as per the methods discussed earlier. The reaction is also called the dimerization of methanol and its general reaction is depicted in Equation (8) [164]. This is the conventional process used on large scale for producing DME as it utilises the same infrastructure used for methanol production [165]. The DME fuel mixture typically has a composition of DME, methanol, and water, where the fuel-grade DME is of its purest form with >99% of DME in the mixture, while technical grade DME has a lower concentration of DME in the mixture [163][166]. As the name suggests, the fuel grade DME is typically used as fuel in the transportation sector, while the technical grade DME is used as an intermediate in the production of other chemicals and hydrocarbon fuels [163][167]. It has promising applications, ranging from being used as a transportation fuel or a solvent in propellants to being used as a direct fuel in fuel cells (DDMEFC) [163][168]. However, its usage in fuel cells is in its early laboratory stages.
Oligomers of DME are also synthetic fuels that are comparable to DME. They are a group of oxymethylene ethers (OMEx), where the term oligomer implies that OMEx is a chain of repeating units of the DME ester [169]. These OMEx have similar properties to DME but have higher molecular mass contributing to significant changes in their physical properties such as higher boiling points or different states at lower temperatures [170]. Mentioning OMEx here is significant since DME has properties similar to that of LPG, while OMEx behaves similarly to LPG but has physical and chemical properties similar to that of diesel [170]. OME of different oligomer lengths is chosen based on the use and need. Coming to the nomenclature, DME is considered OME0, whereas OME1 would imply that one more ester block is added to the initial DME block. For OME2, two extra ester blocks would be added to the chain and so on for other oligomers. Collectively, OMEx is known as polyoxymethylene dimethyl ethers. Figure 12 illustrates the structure of DME and OMEx with an example. OMEx can be prepared from DME through two routes, as illustrated in Figure 13.
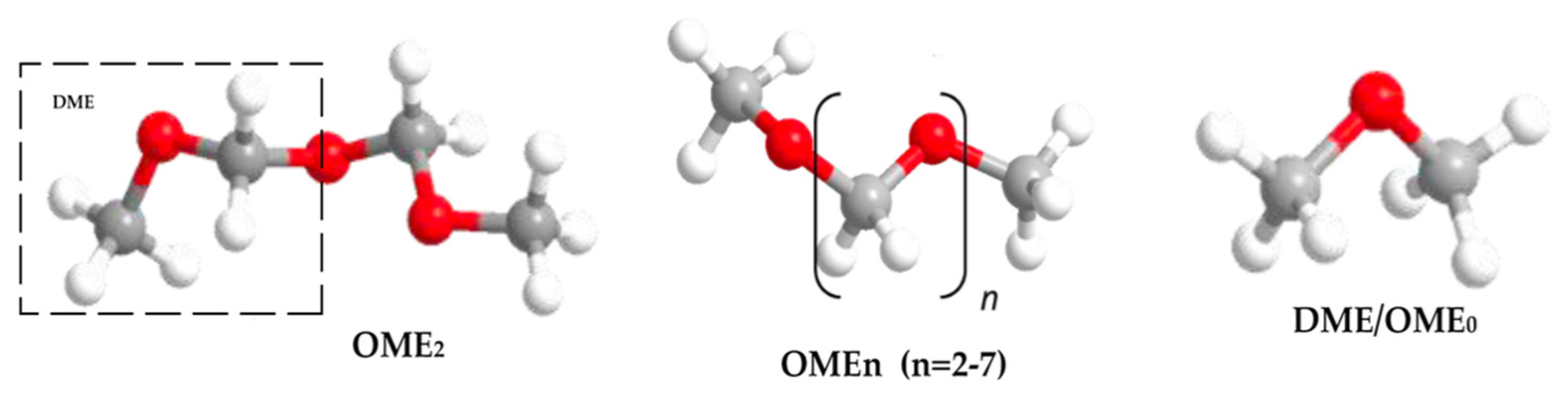
Figure 12. Nomenclature of OMEx.
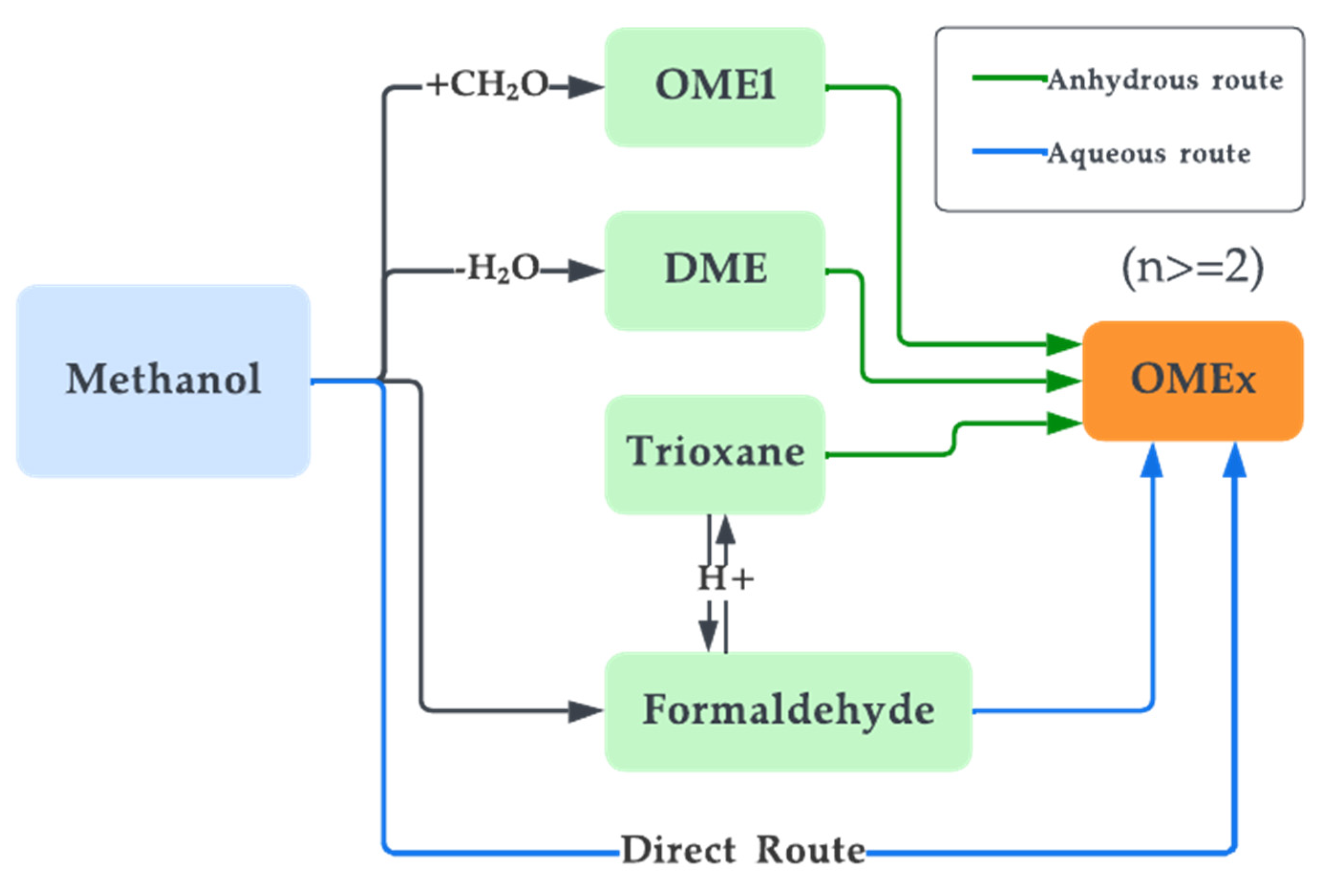
Figure 13. OMEx synthesis routes.
DME can thus be used in a variety of applications such as fuel for heating, transportation, and electricity generation. DME can also be converted into other chemicals and fuels [171]. For example, it can be converted into dimethyl sulphate (DMS), which is used in the production of detergents and other household products. It has a high cetane number and is currently considered for replacing diesel as fuel for trucks and ships. It is thus considered a fuel with significant potential [172]. DME carries similar disadvantages to that of methanol, stopping its large-scale adoption.
5. Power-to-Liquid (PtL)
PtL fuels are a type of synthetic fuel produced through the conversion of electricity into a liquid form. One of the key differences between PtL fuels and other synthetic fuels is the source of the starting material. While other synthetic fuels are typically derived from biomass or other fossil fuels by-products, PtL fuels are produced from renewable electricity. This means that PtL fuels do not release additional carbon dioxide into the atmosphere during their production, making them a more environmentally friendly option compared to traditional fossil fuels. There are two major production pathways in the PtL fuels that are discussed in detail in the upcoming sections, namely, the Fischer–Tropsch route and the methanol route
[173]. The hydrogen required as the starting point for these fuels is typically produced through the electrolysis of water, a process described in earlier sections
[174][175][176]. The hydrogen can then be combined with carbon dioxide to produce a variety of liquid fuels, such as methanol, ethanol, and synthetic diesel, as mentioned in the previous sections. There are several types of PtL fuels that are currently being developed and tested:
-
Hydrogen: It is considered a PtL fuel since it can be produced purely from renewable electricity and water as its feedstock. It can be used as a standalone fuel in fuel cell vehicles or blended with natural gas to produce a lower-carbon fuel for use in internal combustion engines.
-
Methanol, Ethanol and DME: The production of methanol, ethanol, and DME are discussed in the earlier sections in detail, which brings ambiguity in mentioning them here again. With the other feedstocks remaining the same, if the hydrogen required for their production is obtained from clean energy, they are considered as PtL fuels. The production methods, however, remain the same. Methanol and ethanol produced as PtL fuels are also called e-methanol and e-ethanol.
-
Synthetic diesel: Synthetic diesel is a PtL fuel that can be used in any diesel engine with little or no modification.
-
PtL-kerosene: This is an e-fuel produced through renewable energy that is under development stages in the aviation industry.
-
Ammonia: Ammonia is a chemical compound that can be produced from renewable electricity and used as fuel in specialised engines or fuel cells.
One of the main benefits of PtL fuels is their ability to store excess renewable electricity for use when it is needed [177][178]. This can help to smooth out fluctuations in the electricity grid and make it easier to integrate renewable energy sources into the energy mix [179]. PtL fuels also have the potential to reduce greenhouse gas emissions, as they do not release additional carbon dioxide during their production or use [180]. PtL fuels are a promising technology that could help to reduce the reliance on fossil fuels and lower greenhouse gas emissions, especially in the transportation sector [181][182]. While further research and development are needed to bring these fuels to market, they have the potential to play a significant role in the transition to a more sustainable energy future.
5.1. Synthetic Diesel
Synthetic diesel production can be categorised as PtL or GtL. The synthetic diesel discussed in this section is PtL diesel, which is a synthetic fuel that is produced by converting H
2 and CO
2 into liquid hydrocarbons. It is also important to note that this is a hydrocarbon, while biodiesel is an ester. This implies that this is essentially the same as HVO, except synthetic diesel is obtained through a different chemical process. It is produced through a process known as the Fischer–Tropsch process, which involves combining hydrogen and carbon monoxide to form a variety of liquid hydrocarbons, including diesel, gasoline, and jet fuel, as shown in
Figure 9 [183]. The production of PtL diesel typically involves the use of electrolysis to produce hydrogen from water, which is then combined with carbon dioxide to form syngas and later, into this synthetic fuel. The carbon dioxide can be sourced from a variety of sources, including industrial emissions and biogas. PtL diesel has high energy density and is easy to transport and store, making it suitable for use in a variety of applications
[184]. It is currently used as a replacement for traditional diesel fuel in cars, trucks, and other vehicles, and it can also be used as jet fuel for aeroplanes. It is also used in the production of chemicals and other industrial products.
The Fischer–Tropsch process cannot go unmentioned while talking about synthetic fuels. It is a chemical reaction that is used to convert hydrogen and carbon monoxide into a variety of liquid hydrocarbons, including diesel, gasoline, and jet fuel. It was developed in the 1920s by German chemists Franz Fischer and Hans Tropsch, and it has since been used to produce synthetic fuels from a variety of feedstocks, including natural gas, syngas, and biomass
[185][186]. The Fischer–Tropsch process typically involves the use of a catalyst, such as iron or cobalt, to facilitate the reaction between hydrogen and carbon monoxide
[187]. The reaction takes place in a reactor at high temperatures and pressures, and it produces a range of liquid hydrocarbons with different molecular structures, depending on the specific conditions used.
Figure 14 illustrates the process from input being syngas, as a continuation of
Figure 9. The overall equation for the Fischer–Tropsch process is shown in Equation (9) (where n is the number of carbon atoms in the resulting hydrocarbon).
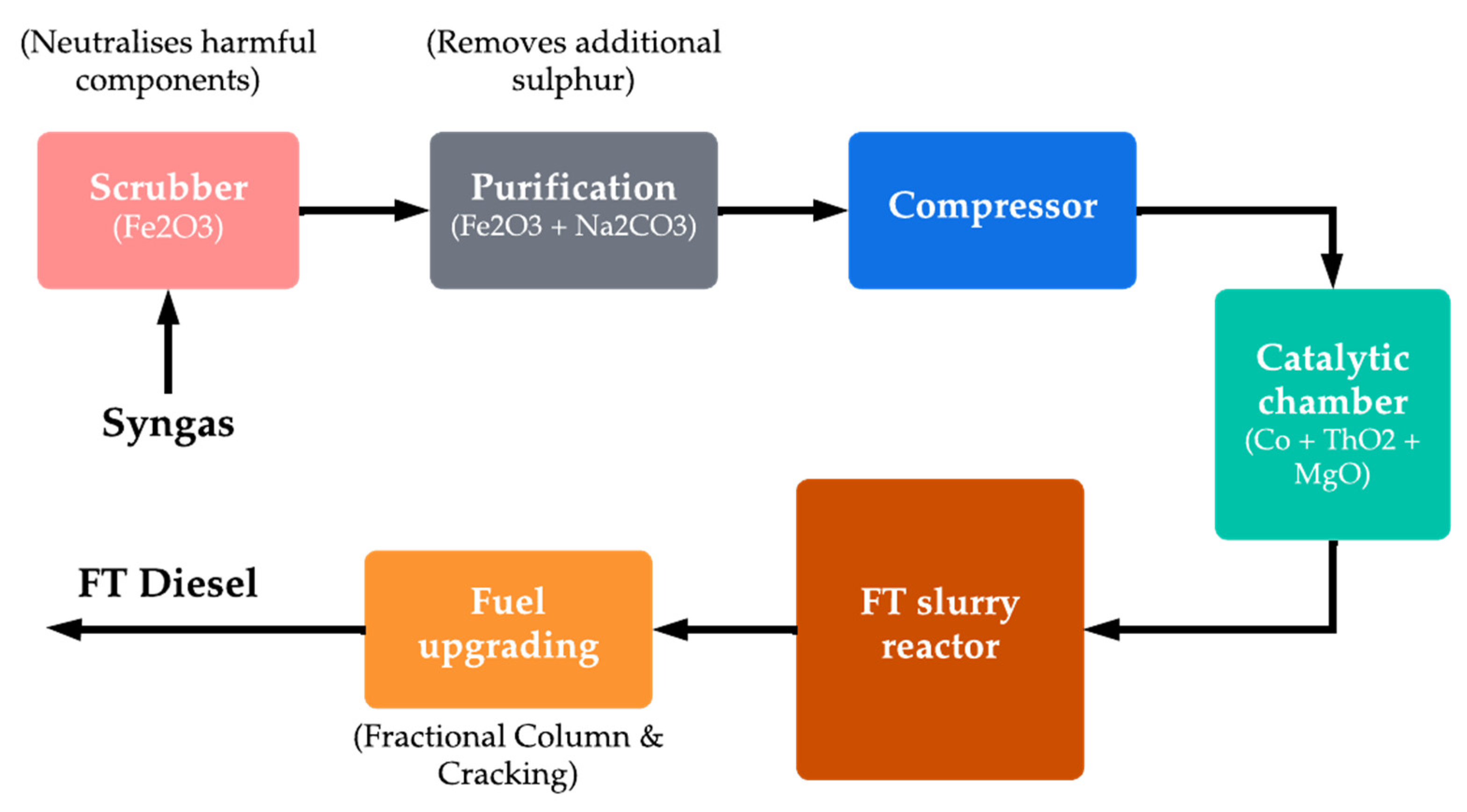
Figure 14. Typical Fischer–Tropsch process for FT diesel production from syngas.
The mentioned process is used in a variety of applications, including the production of synthetic fuels such as synthetic diesel, synthetic gasoline, and jet fuel for aviation, naphtha, and in the production of chemicals and other industrial products [188][189][190]. The method can be employed to produce synthetic fuels at economical costs when compared to the production of synthetic fuels through other methods. However, the process is energy-intensive and can be costly under certain conditions, and the feedstocks used can have significant environmental impacts, depending on their source [190][191]. After obtaining the fuel by Fischer–Tropsch, the excess by-products are processed under the water–gas shift (WGS) reaction [192], which is a chemical reaction in which CO and water are converted into H2 and CO2, which can be once again used as feedstock for the production of other synthetic fuels [193]. Synthetic diesel is considered to be sustainable if the energy required to synthesise the fuel is obtained from renewable sources. As mentioned in earlier sections, the technological limitation of commercial-scale biomass gasification is also the limiting factor for the expansion of FT diesel.
5.2. PtL-Kerosene
PtL-kerosene is another synthetic fuel produced through the Fischer–Tropsch process. The production of PtL-kerosene typically involves the use of electrolysis to produce hydrogen from water, which is then combined with carbon dioxide to form syngas, followed by the Fischer–Tropsch process to produce PtL-kerosene. To improve the quality of its production, a hydrocracker is employed after the FT process to break long hydrocarbon chains and aid the production of hydrocarbons in the middle distillate range, where kerosene resides [194]. PtL-kerosene is considered to be a Sustainable Aviation Fuel (SAF) as its main application is for powering aircraft [176][178][195]. The PtL-kerosene produced is blended with kerosene-based jet fuels such as Jet A and Jet A-1, JP-5, and JP-8. Jet A-1 is the closest form of jet fuel to PtL-kerosene, as it itself is a highly refined form of kerosene [196][197][198]. Figure 15 shows the cycle of PtL-kerosene production and its use.
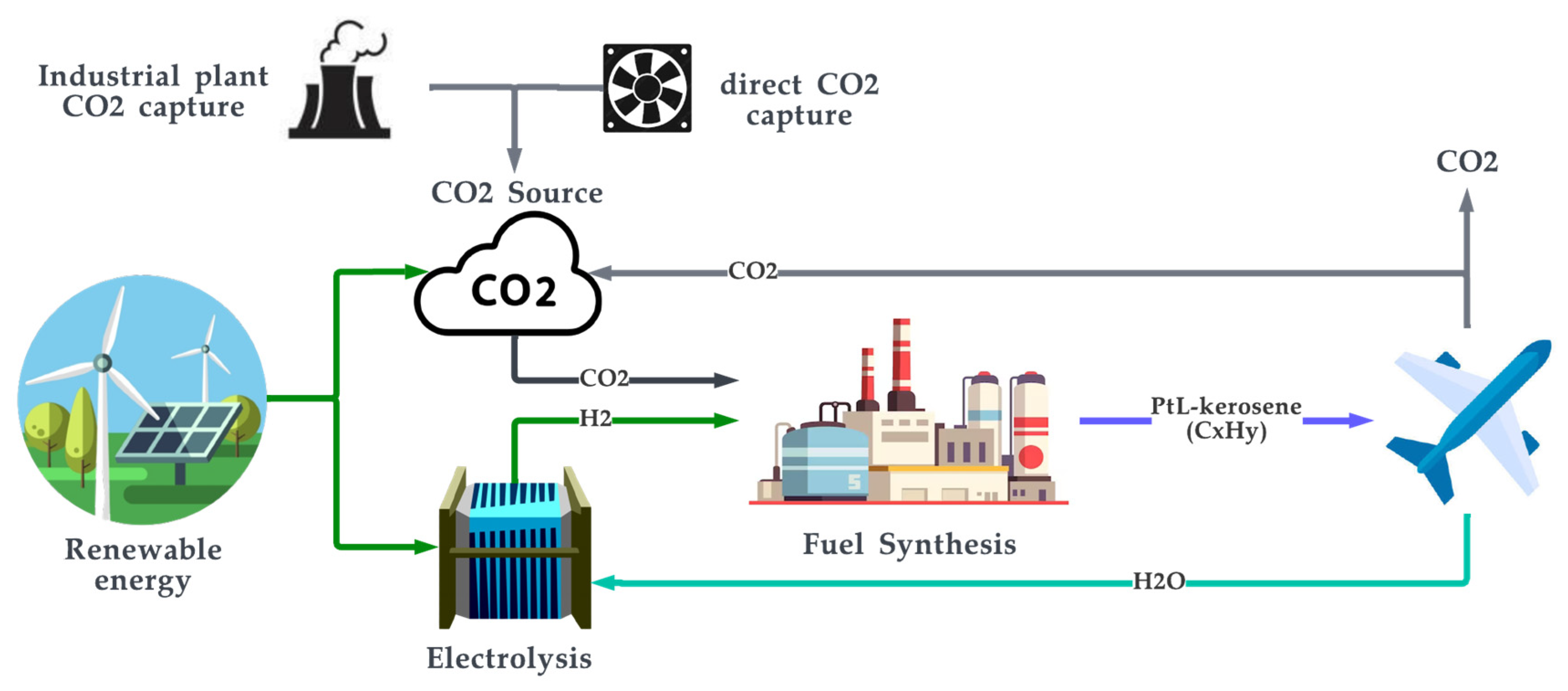
Figure 15. PtL-kerosene production and use cycle.
The production quantity of SAF is negligible compared to conventional aviation fuel production volumes, as the commercial-scale production of this fuel has a few challenges to consider, such as compositional complexity and variability of feedstock, rapid growth in hydrogen generation technologies, and risk in investment of erecting plants considering a history of abandoned flagship projects in the aviation industry [199]. Despite this, its adoption is likely to increase as more companies and countries seek to transition to a cleaner and more sustainable energy system.
5.3. Ammonia
Ammonia is a chemical compound that is widely used in a variety of applications such as in the manufacture of fertilizer, refrigerants, and several other chemicals
[200]. There are several routes for synthesizing ammonia, including electrochemical, thermochemical, and electrolytic routes. Conventionally, it is produced through the Haber–Bosch process, which involves the reaction of nitrogen and hydrogen at high temperatures and pressures
[201]. Compared to the Haber–Bosch process, the electrochemical route is significantly underdeveloped but carries the potential of consuming lower energy to produce ammonia
[201]. Ammonia is classified as an “electro-fuel” since it can be created from nitrogen (captured from the air) combined with H
2 (produced through electrolysis). As with any other PtL fuel, the electricity necessary for electrolysis is produced using renewable energy sources. Ammonia has a high energy density, resulting in a growing interest in using ammonia as a synthetic fuel. It is being researched as a synthetic fuel, primarily for marine transportation, but also for cars and buses
[202][203]. Additionally, it can also be used as a chemical feedstock in various industrial processes, such as the production of chemicals, fertilizers, and steel, and can also be used for electricity generation by burning it in a fuel cell. However, it has a few downsides, such as poor calorific value, low-cetane value, and low-flame speed, which make it challenging for use in combustion engines
[204]. They are also of a toxic nature, which can easily contaminate their surroundings; thereby, it requires cautious usage
[205].
Ammonia cracking is another advantage of ammonia. Ammonia is broken down into smaller molecules, such as hydrogen and nitrogen, through the application of heat and pressure. This process is a type of pyrolysis. Ammonia cracking is typically carried out in a reactor or furnace, where it is heated to a high temperature (typically around 400–600 °C) and pressure (usually around 30–100 atm)
[206]. At these conditions, the bonds between the atoms in the ammonia molecule become weaker and can be broken, resulting in the formation of smaller molecules. This process is often used to produce hydrogen, which is a valuable chemical feedstock and an important fuel for fuel cells. The applications of ammonia are pictorially illustrated in
Figure 16. The major disadvantage of ammonia is its toxic nature which can be detrimental to the environment
[207].
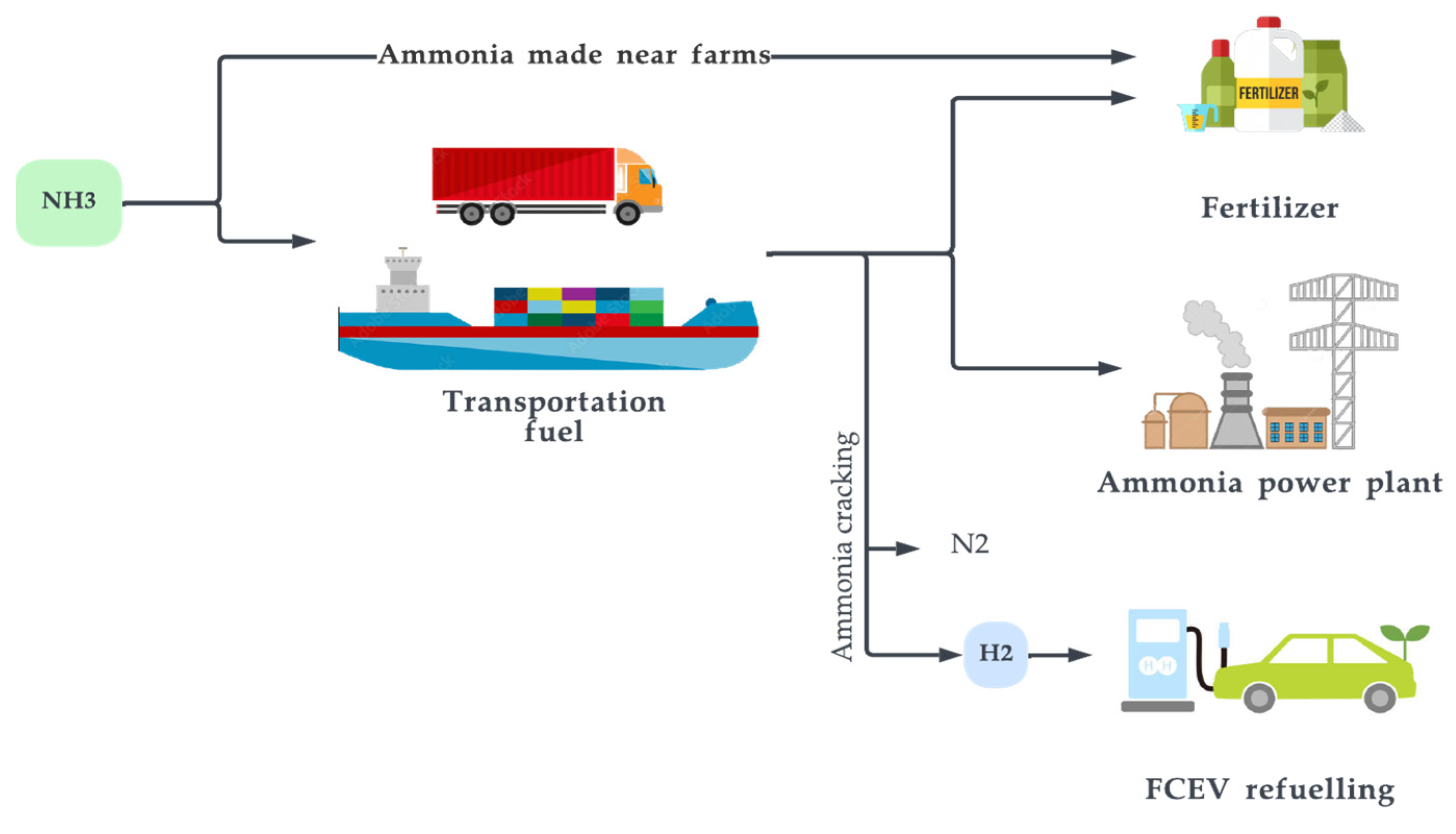
Figure 16. Major uses of ammonia.
6. Gas-to-Liquid (GtL)
GtL fuels are a type of synthetic fuel produced through the conversion of natural gas or other hydrocarbon gases into liquid form. These fuels have gained significant attention in recent years as a way to extend the use of natural gas beyond electricity generation and heating applications. One of the key differences between GtL fuels and other synthetic fuels is the source of the starting material. While other synthetic fuels are typically derived from fossil fuels, GtL fuels are produced from natural gas or other hydrocarbon gases, such as ethane, propane, butane, or syngas, which are commonly found in natural gas deposits
[208]. This means that GtL fuels can potentially contribute to greenhouse gas emissions depending on the source of the natural gas and the efficiency of the GtL process. The conventional natural gas extraction method involves drilling into underground natural gas deposits and extracting the gas through a well. The unconventional natural gas production method involves extracting natural gas from shale formations or coal beds using techniques such as hydraulic fracturing (fracking) or coal bed methane recovery
[209]. Following the extraction of the source, the production of GtL fuels is essentially carried out through the same technical processes described in detail in the earlier sections.
Waste management is another global challenge faced across developing and developed countries. Biogas production is another method employed to produce these gases through the anaerobic digestion of organic matter from food waste or agricultural waste, thus offering an advantage that no other feedstock in the entire list of synthetic fuel feedstock serves. Anaerobic digestion is a biological process that occurs in the absence of oxygen and involves the breakdown of organic matter by microorganisms
[210]. The process of biogas production through anaerobic digestion typically occurs in a sealed container called a digester. The organic matter, which can include agricultural waste, food waste, or other biomass, is mixed with water and allowed to decompose in the absence of oxygen. As the organic matter breaks down, microorganisms produce methane and carbon dioxide, which are captured and stored in the digester
[210]. Several different types of anaerobic digestion systems can be used to produce biogas, including batch systems, continuous flow systems, and plug flow systems. The specific type of system that is used will depend on the specific feedstock and the desired biogas production rate. This is considered to be the most appropriate waste management technique for the developing world
[211][212][213].
After obtaining the feedstock, the production processes of this type of fuel are the same as explained in the earlier sections. Synthetic fuels from GtL feedstock are typically produced through the Fischer–Tropsch process, which requires the feedstock gas to be first converted into syngas. The syngas is then converted into a variety of liquid GtL fuels. Several types of GtL fuels are currently being produced and used:
-
GtL Diesel: Gas-to-liquid (GtL) diesel and power-to-liquid (PtL) diesel are both essentially the same synthetic diesel fuel produced through different processes. One key difference between GtL and PtL diesel is the source of the starting material. GtL diesel is produced through the conversion of natural gas or other hydrocarbon gases into a liquid form, while it is considered PtL when the energy required is obtained from renewable sources. As a result, PtL diesel is considered to be a more environmentally friendly option compared to GtL diesel, as it does not release additional carbon dioxide into the atmosphere during its production. GtL diesel is commonly used in transportation and other applications and is often considered to be a cleaner burning fuel compared to traditional diesel, as it contains fewer impurities and produces fewer emissions.
-
Jet fuel: Jet fuel is another type of GtL fuel that is commonly used in the aviation industry. GtL jet fuel is often considered to be a more sustainable alternative to traditional jet fuel, as it has a lower carbon intensity and produces fewer emissions. This is the same as the SAF discussed in the earlier sections and the differentiation between them is once again analogous to that of synthetic diesel in the earlier point.
-
Naphtha: Naphtha is a type of GtL fuel that is used as a feedstock for the production of chemicals and plastics. It can be produced from other hydrocarbon gases and is sometimes used as a blend stock for gasoline.
-
LPG: LPG, or liquefied petroleum gas, is a type of GtL fuel that is commonly used for heating purposes. It is a mixture of propane and butane and is often considered to be a cleaner-burning alternative to traditional fossil fuels.
GtL fuels are a promising technology that can help to extend the use of natural gas beyond electricity generation and heating applications. While GtL fuels can potentially contribute to greenhouse gas emissions depending on the source of the natural gas and the efficiency of the GtL process, they are generally considered to be cleaner-burning fuels compared to traditional fossil fuels. As research and development in this area continue, GtL fuels are also likely to play an increasingly important role in the energy mix.
6.1. Naphtha
Naphtha is a type of liquid hydrocarbon that is produced as a by-product while refining crude oil in the petrochemical industry [214]. It is a colourless or light-yellow liquid with a gasoline-like odour and is a complex mixture of hydrocarbons that can be used as a feedstock for the production of various chemicals and fuels. Naphtha is considered a GtL fuel since it can be produced through the FT process discussed in earlier sections [215]. However, on an industrial scale, it typically is produced through a refining process known as distillation [216]. First, crude oil or coal tar is heated and distilled to separate it into various components based on their boiling points [217]. The resulting mixture is then further processed through a series of distillation columns, where the naphtha is separated from other components such as gasoline and kerosene. It is typically produced from the middle distillates of crude oil, which are the components that have a boiling point between approximately 200–300 °C. The naphtha is then treated with hydrogen and passed over a catalyst, such as a metal or metal oxide, to remove impurities and improve its quality. The purified naphtha is then cooled and stored for further use or transportation to other facilities for further processing. Naphtha is typically composed of hydrocarbons with carbon numbers in the range of about 5 to 12 [218]. It is made up of n-paraffins and olefins; the paraffin yield quality favours high temperature and low pressure while the olefin composition varies on the reaction pathway, where a greater olefin composition denotes a higher octane number and, consequently, better fuel quality [219][220]. During the refining process of petroleum, crude oil is heated and distilled to separate it into various hydrocarbon components based on their boiling points. In addition, bio-naphtha is a type of naphtha that is produced from biomass feedstocks rather than fossil fuels such as petroleum or coal. Similar to the typical FT process, bio-naphtha can be produced through a biomass-to-liquid Fischer–Tropsch (BTL FT) process [221]. Figure 17 depicts the cycle of naphtha’s applications.
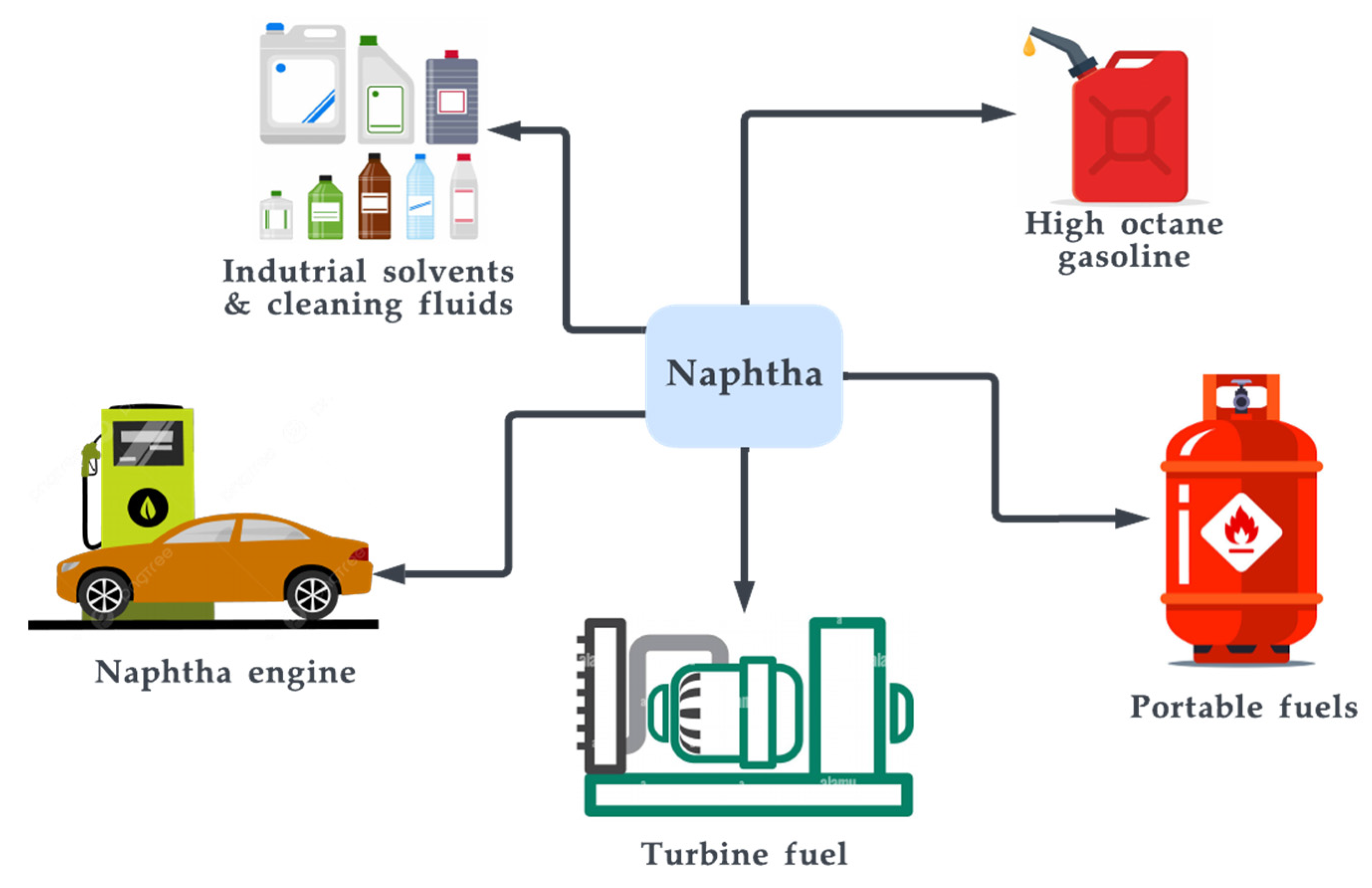
Figure 17. Applications of naphtha.
Naphtha has a wide range of uses in the chemical and fuel industries. It is commonly used as a feedstock for the production of gasoline, as well as for the production of other chemicals such as petrochemicals, solvents, plastics, and resins [222]. Naphtha is also used as fuel for portable stoves and other heating and cooking applications. One of the key properties of naphtha is its high energy density, which makes it an attractive fuel for transportation applications [223][224]. On the other hand, it is important to note that the production and use of naphtha, like other fossil fuels, can have environmental impacts. However, the environmental impacts of naphtha can be mitigated through the use of advanced technologies and practices. It is also important to be aware that naphtha can have potentially harmful effects on human health if contacted directly.
6.2. Liquefied Petroleum Gas (LPG)
LPG is a mixture of propane and butane and is produced through the refining of petroleum or the extraction of natural gas [225]. It is a GtL fuel that is produced through several steps involving the conversion of natural gas or other gases into liquid form. First, natural gas or other gases are cleaned and purified to remove impurities such as water and sulphur. The purified gases are then converted into synthesis gas, or syngas, through a process called steam methane reforming. This process involves the reaction of the gas with steam and a catalyst to produce hydrogen and carbon monoxide. The syngas is then passed over a catalyst, such as a metal or metal oxide, to convert it into a range of hydrocarbons, including LPG [226]. LPG is also produced through fractional distillation as explained in the previous section, with it being in the top tier as shown in Figure 18. The parameters that favour a high yield of LPG include lower temperature, optimum steam-to-feed ratio and more trays in the furnace [227].
LPG has a wide range of applications, including as a fuel for cooking and heating in residential and commercial settings, where it is a popular choice due to its clean-burning nature, which results in fewer emissions compared to other fossil fuels [228]. It is also used as fuel for vehicles, including cars, buses, and trucks. It is also used as feedstock for the production of chemicals and plastics and as a solvent for a variety of products. It is commonly used in the production of propylene, which is used in the manufacture of plastics, resins, and other products [229]. An uncommon usage of LPG is its usage as a fuel for recreational vehicles, such as boats and recreational vehicles (RVs). It is also a relatively inexpensive fuel, making it an attractive option for many consumers [230]. It is abundant in many parts of the world but is often difficult to transport due to its gaseous nature [231]. Its transportation difficulty, higher engine burning temperatures, higher consumption, NOx emission, non-sustainable nature, and high cost of equipment are its major drawbacks [232]. Although LPG is not sustainable, it has a significantly lower impact on the environment as opposed to other fossil fuels.
7. Current challenges and the Future Landscape of Synthetic Fuels
Governments across the world have laid out roadmaps and targets for achieving net zero carbon emissions to address the impacts of climate change and reduce the risk of its negative consequences. These efforts are being monitored by organisations such as the United Nations Framework Convention on Climate Change (UNFCCC), the International Energy Agency (IEA), and the Intergovernmental Panel on Climate Change (IPCC). To discuss progress towards these targets and share ideas and best practices, several conferences take place annually, including the United Nations Climate Change Conference (COP), the Global Summit on Climate Action (GSCA), and the Carbon Pricing Leadership Coalition (CPLC) Annual Conference. Developed countries like the US, the EU, the UK, Japan, Australia, and so on have set 2050 as their net zero targets while developing countries like China and India have set their targets at 2060 and 2070, respectively. These efforts demonstrate a commitment to transitioning to a more sustainable and low-carbon future. The major barriers for countries transitioning to net zero emissions targets include:
-
Cost: Transitioning to low-carbon or zero-emissions technologies can be expensive. Many countries rely heavily on fossil fuels for energy, and transitioning away from these sources will require significant investments in new infrastructure.
-
Technological challenges: There may be technical challenges involved in transitioning to low-carbon or zero-emissions technologies, such as the need to develop new technologies or modify existing ones.
-
Political challenges: There may be political challenges to transitioning to net zero emissions, as it may require significant policy changes and the implementation of new regulations. This can be difficult to achieve, particularly if there is resistance to change from certain groups or sectors of society.
-
Behavioural change: Achieving net zero emissions will also require individuals and businesses to change their behaviour, such as using low-carbon transportation options and adopting energy-efficient practices. Changing behaviours can be difficult, particularly if people are resistant to change.
-
International cooperation: Achieving net zero emissions will require cooperation between countries, as global emissions need to be reduced. This can be challenging, as different countries have different priorities and may not agree on the best course of action.
The challenges in adopting synthetic fuels are the high cost of production, lack of infrastructure, consumer awareness, and finally, the finance required to adopt them amongst developing countries. Synthetic fuels are currently much more expensive to produce than fossil fuels, making them less attractive to consumers and producers. They require specialised storage and distribution systems, which may not be in place in many areas. The lack of consumer awareness and understanding is making adoption difficult. However, plans have been announced to implement subsidies for synthetic fuels as part of energy transition and decarbonisation efforts. These subsidies include incentives to support the development and deployment of synthetic fuels, including funding for research and development, tax incentives, and grants for pilot projects. They are typically designed to reduce the cost of producing synthetic fuels—especially hydrogen—and to encourage the development and deployment of these technologies. Hydrogen, in particular, is the most subsidised synthetic fuel because it has the potential to play a significant role in the transition to a low-carbon energy system. Hydrogen can be produced from a variety of feedstocks and is also the feedstock for other synthetic fuels. Hydrogen also carries a huge potential compared to all existing fuels (fossil and synthetic) with respect to its high specific energy. A comparison of specific energies in Lower Heating Value (LHV) is depicted in
Figure 18 [72][170][233][234][235][236]. There are several different types of synthetic fuels that can be produced, and the production processes for these fuels can vary widely. However, many synthetic fuel production processes are interconnected in the sense that they often rely on similar raw materials, share the same initial stages of production, and may use similar types of catalysts or other chemical processes. Additionally, certain fuels are produced from other fuels as feedstock. Most of the processes are interlinked, and an overview of all the explained production processes is illustrated in
Figure 19.
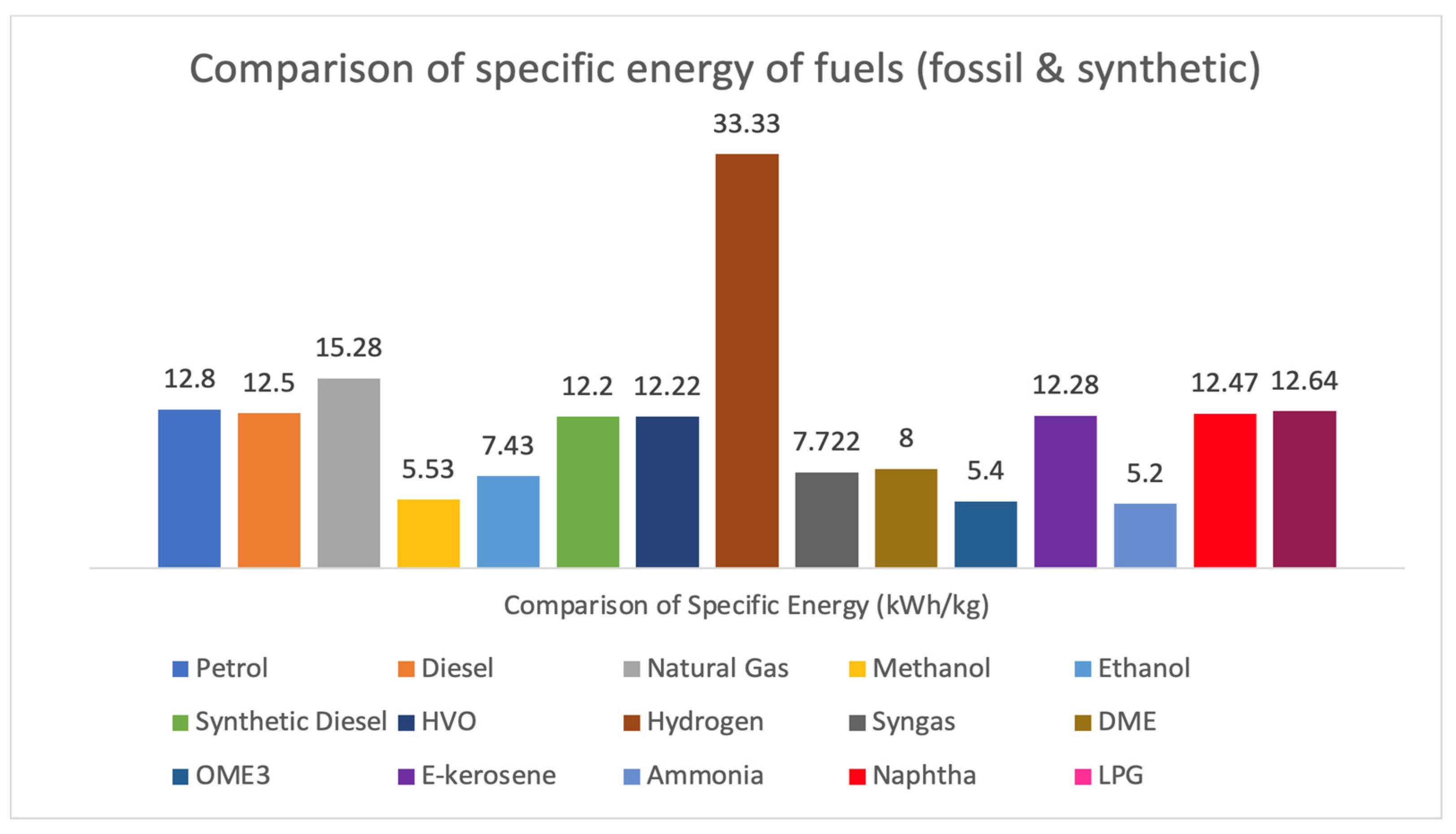
Figure 18. Fuel comparison chart.
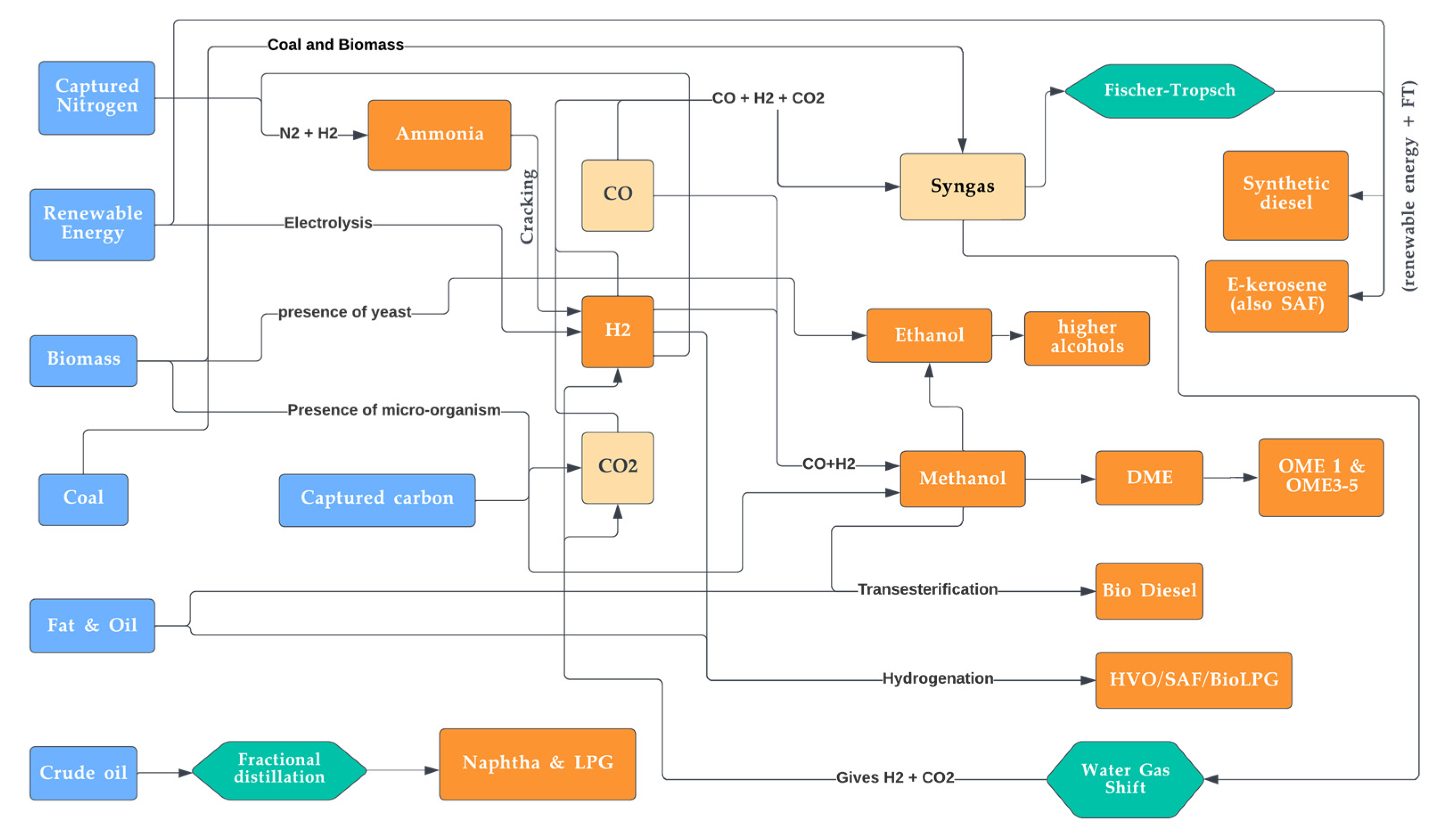
Figure 19. Overview of combined routes for the synthesis of various major synthetic fuels.
Several surveys and studies are ongoing to predict the role and growth of synthetic fuels in a global net-zero economy. For example, the International Energy Agency (IEA) has predicted that biofuels, including synthetic biofuels, will play a significant role in decarbonizing the transportation sector
[237][238]. Similarly, the Energy Transition Commission (ETC) has predicted that the shipping industry will increasingly turn to synthetic fuels, such as biofuels and hydrogen-based fuels, in order to meet emissions reduction targets. The market for synthetic fuels in the aviation industry will grow significantly in the coming years, as the report suggests there is sufficient biomass to decarbonise the aviation industry
[239]. Another report by the International Energy Agency (IEA) estimates that hydrogen and e-fuels could provide up to a quarter of the energy needed to achieve net zero global emissions by 2050 with renewable energy prices dropping by 30% by 2030
[240].
However, it is difficult to predict exactly what percentage of energy hydrogen and e-fuels will provide in a net zero global economy, as this will depend on a variety of factors, including technological developments, availability of raw materials, economic conditions, and policy decisions. The increase in demand for sustainable and decarbonised energy sources is one of the key drivers of this growth and its essential to consider each and every path towards sustainability. The demand fundamentally owes to their capacity to be created from waste materials, giving synthetic fuels the potential to become a more competitive alternative to conventional fossil fuels in the future. They could offer a low-carbon energy source in addition to aiding in waste management and utilisation. However, the significantly lower efficiencies of synthetic fuels in comparison to electric-based counterparts (such as EVs), along with their negligible market capture in opposition to the dominance of electric-based systems, question the future of their large-scale adoption. Synthetic fuels might perhaps be utilised for energy generation to fulfil the energy needs of electric-based systems. They may additionally fill in the gaps of applications where electric-based systems are not feasible, such as marine transportation.