Circadian rhythms, as biological rhythms with a period of approximately 24 h, are regulated and controlled by an endogenous time-keeping mechanism, i.e., the circadian clock
[35][36][37][38]. In mammals, the central clock is situated at the suprachiasmatic nuclei (SCN) of the anterior hypothalamus
[39]. The mammalian SCN neurons exhibit higher activity in electrical physiology and metabolism during the daytime
[40][41]. External light is received by intrinsically photosensitive retinal ganglion cells (ipRGCs) and transmitted to the SCN via the retinohypothalamic tract (RHT)
[42]. The efferent from the SCN projects to the pineal gland and drives the rhythmic release of melatonin, a sleep-promoting hormone. Since light is known to inhibit melatonin synthesis, the daily oscillation of melatonin is similar in both diurnal and nocturnal animals
[43][44] and helps to synchronize peripheral organs in the body
[45]. Intriguingly, most organs, tissues, and cells display circadian rhythmicity, regulated by the local peripheral clock, as well as neural, hormonal, and metabolic cues from the SCN
[46].
Three transcription-translation feedback loops as molecular time-keeping mechanisms are known to generate, regulate, and maintain circadian rhythms
[47][48]. In the primary loop, the CLOCK: BMAL1 heterodimer as the positive limb activates the expression of target genes, including
Per genes (
Per1,
Per2, and
Per3 ) and
Cry genes (
Cry1 and
Cry2) via binding to E-box (5′-CACGTG-3′) and E’-box (5′-CACGTT-3′) in their promoter regions
[49], whereas the PER: CRY heterodimer as the negative limb interferes with the transcriptional activity of the CLOCK-BMAL1 heterodimer and turns off their expression
[50]. In the second loop,
Rorα/β and
Rev-erbα/β are regulated by CLOCK and BMAL1 via E-box, whereas their proteins RORα/β and REV-ERBα/β activate and suppress
Bmal1 by competing for binding to the RORE (retinoic-acid-related orphan receptor response element) in the
Bmal1 promoter region (
Figure 1). In the third loop, D-box-containing proline and acidic amino-acid-rich basic leucine zipper (PAR bZip) genes
Dbp (albumin D-box-binding protein),
Hlf (hepatic leukemia factor),
Tef (thyrotroph embryonic factor), and
E4bp4/Nfil3 (E4 promoter-binding protein 4/nuclear factor interleukin-3-regulated protein/nuclear factor, interleukin 3 regulated) are all regulated by CLOCK and BMAL1 via E-box, whereas their proteins DBP, HIF, TEF, and E4BP4/NFIL3 bind to D-box in the promoter regions of their target genes, where DBP, HIF, and TEF activate D-box-containing genes and E4BP4/NFIL3 represses them
[51]. Among these three circadian-clock-controlled
cis-elements-mediated transcriptional feedback loops, the E/E’-box-mediated loop plays the dominant role in the circadian clock
[52]. However, the E/E’-box-mediated loop, combined with the RORE-mediated loop and the D-box-mediated loop, forms the necessary transcriptional repression and delays for oscillating approximately 24 h a day. In particular, E/E’-box, D-box, and RORE act in the morning, evening, and night, respectively
[53][54]. In addition, the mechanistic/mammalian target of the rapamycin (mTOR) pathway, implicated in numerous neurological disorders, has been shown to contribute to circadian regulation
[55][56] by activating circadian clock genes through the phosphorylation of the translation factor S6K1
[55][57][58]. Specifically, S6K1 phosphorylates GSK3β, which, in turn, phosphorylates CLOCK, BMAL1, and REV-ERB
[59].
Figure 1. Mammalian circadian clockwork model. Three transcription-translation feedback loops are known to operate in the mammalian circadian clock. In the first loop, the CLOCK: BMAL1 heterodimer activates the expression of target genes, including Per genes (Per1, Per2, and Per3) and Cry genes (Cry1 and Cry2) via binding to E-box (5′-CACGTG-3′) in their promoter regions, whereas the PER: CRY heterodimer interferes with the transcriptional activity of the CLOCK-BMAL1 heterodimer and turns off their own expression. In the second loop, Rorα/β and Rev-erbα/β are regulated by CLOCK and BMAL1 via E-box, whereas their proteins RORα/β and REV-ERBα/β activate and suppress Bmal1 by competing for binding to the RORE (retinoic-acid-related orphan receptor response element). In the third loop, Dbp, Hlf, Tef, and E4bp4/Nfil3 are all regulated by CLOCK and BMAL1 via E-box, whereas their proteins DBP, HIF, TEF, and E4BP4/NFIL3 bind to D-box in the promoter regions of their target genes, where DBP, HIF, and TEF activate D-box-containing genes and E4BP4/NFIL3 represses them. Among these three circadian clock-controlled cis-elements-mediated transcriptional feedback loops, the E/E’-box-mediated loop plays the dominant role in the circadian clock. In addition, the mTOR pathway has been shown to contribute to circadian regulation. Color arrows indicate transcription or translation, black arrows transcription activation, and turnstile symbol suppression.
4. The Roles of Circadian Clock Genes in Epilepsies
Two mechanisms have been proposed to account for the effects of the circadian clock on seizures
[4]: one is that canonical clock genes such as
BMAL1 and
CLOCK contribute directly to epilepsies, and the other is that the circadian clock acts through certain signaling pathways to exert its effect on epilepsy. Loss of the circadian PAR bZip transcription factors DBP, HIF, and TEF resulted in lethal spontaneous epileptic seizures in mice
[51]. TEF was shown to regulate the expression of pyridoxal kinase involved in converting B6 vitamers into pyridoxal phosphate (PLP)
[51], and downregulation of PLP is associated with the susceptibility of seizures
[60]. The CLOCK protein was revealed to be significantly downregulated in the neurons of human focal epilepsy patients, and the seizure threshold was reduced in excitatory pyramidal neuron-specific
Clock−/− knockout mice. Similarly, downregulation of BMAL1 was found in hippocampal sclerosis (HS) patients with HS International League Against Epilepsy (ILAE) type I and III
[61], and the threshold of seizures was also reduced in
Bmal1−/− knockout mice
[47]. REV-ERBα was shown to be upregulated in the brain tissues of epileptic human patients and mice, while downregulation of
Rev-erbα in mice reduced their seizure susceptibility, and REV-ERBα activated GABA transporters
Slc6a1 (
Gat1) and
Slc6a11 (
Gat3) through repressing
E4bp4/Nfil3 to downregulate GABA signaling
[62].
5. Mutual Effects between Epilepsy and Sleep
Sleep and epilepsy are reciprocally affected. On the one hand, NREM sleep, especially NREM stage 1 (N1) and stage 2 (N2) sleep, facilitates epileptogenesis, while REM sleep inhibits it [63]. Specifically, REM sleep has the most suppressive effect during the EEG desynchronization period [64], whereas NREM sleep facilitates seizures due to the effect of the synchronous discharge of the thalamocortical network [65][66], as evidenced by the fact that 95% of seizures occur in NREM sleep [67]. An interesting study demonstrated that a small lesion in focal cortical dysplasia (FCD) type II patients is highly associated with sleep-related epilepsy [68]. Interictal epileptiform discharges (IEDs) have been used to evaluate seizure exploding [69]. Melatonin, a sleep-promoting hormone, appears to contribute to the nocturnal predominance of IEDs during sleep [70], and reduced melatonin levels with shifted phases were reported in epilepsy patients [71][72][73]. In adult patients, frontal lobe epilepsy is the archetypal sleep-related epilepsy that tends to occur during sleep, whereas juvenile myoclonic epilepsy often occurs in the morning [74].
On the other hand, epileptic activity has been shown to affect sleep continuity, which increases waking time after sleep onset, reduces REM sleep quality, and delays the first REM sleep episode in epilepsy patients [75]. Epilepsy patients often suffer from severe sleep disturbances such as excessive daytime sleepiness, sleep fragmentation, and insomnia [76]. Nocturnal seizures may lead to severe sleep fragmentation and even NREM parasomnia [77], and diurnal seizures also result in the alteration of the sleep architecture [78]. The epileptic activity also alters the sleep oscillations, likely through desynchronizing hippocampal IED and remote cortical spindles [79]. The sleep architecture of JME patients is severely altered with prolonged REM onset latency and decreased REM percentage [80].
Hence, sleep problems are prevalent among epilepsy patients, and reciprocal interactions between epilepsy and sleep should be underscored in epilepsy treatment. Various questionnaire-based instruments, such as the Pittsburgh Sleep Quality Index (PSQI) and the Sleep Condition Indicator, should be employed to evaluate the sleep status of patients, and possible comorbid sleep disorders also must be assessed. In particular, comorbid sleep disorders, once verified, should be treated separately. Generally, after epileptic patients are cured with drug treatment or surgeries, their sleep quality is expected to be improved with normal sleep patterns and melatonin levels [74]. Good quality of sleep helps contain seizures.
6. A Chronomodulated Strategy for Epilepsy Therapy
6.1. Circadian Mechanisms Underlying Epileptogenesis
Although our understanding of the mechanisms underlying epilepsy remains limited, mounting evidence indicates circadian involvement in epilepsy pathogenesis, as numerous types of human epilepsies display robust daily rhythmicity [21]. Future efforts will aim to identify circadian biomarkers by elucidating molecular genetic mechanisms underlying how the circadian clock regulates the robust rhythmicity of these epilepsies. In doing so, the circadian clock system and possible circadian-clock-regulated epilepsy processes such as the hypothalamus–pituitary–adrenal (HPA) axis and the hypothalamus–pituitary–gonadal (HPG) axis should be investigated. The circadian clock regulates the HPA axis [81][82] and the HPG axis [83][84], which are known to contribute to epilepsy pathogenesis [85][86][87]. In some epilepsies, it would be worthwhile to investigate how the circadian clock acts through the HPA axis or the HPG axis to regulate epilepsy pathogenesis. Furthermore, it would be intriguing to determine whether the core circadian clock genes and circadian-clock-controlled epilepsy genes harbor mutations or whether the normal rhythmic expression patterns of these circadian clock genes and circadian-clock-controlled epilepsy genes are altered in individual patients. This line of investigation should provide insights into the circadian regulation of the dynamics of the pathogenesis of a particular epilepsy, which should provide cues for the time-of-day delivery of AEDs (Figure 2).
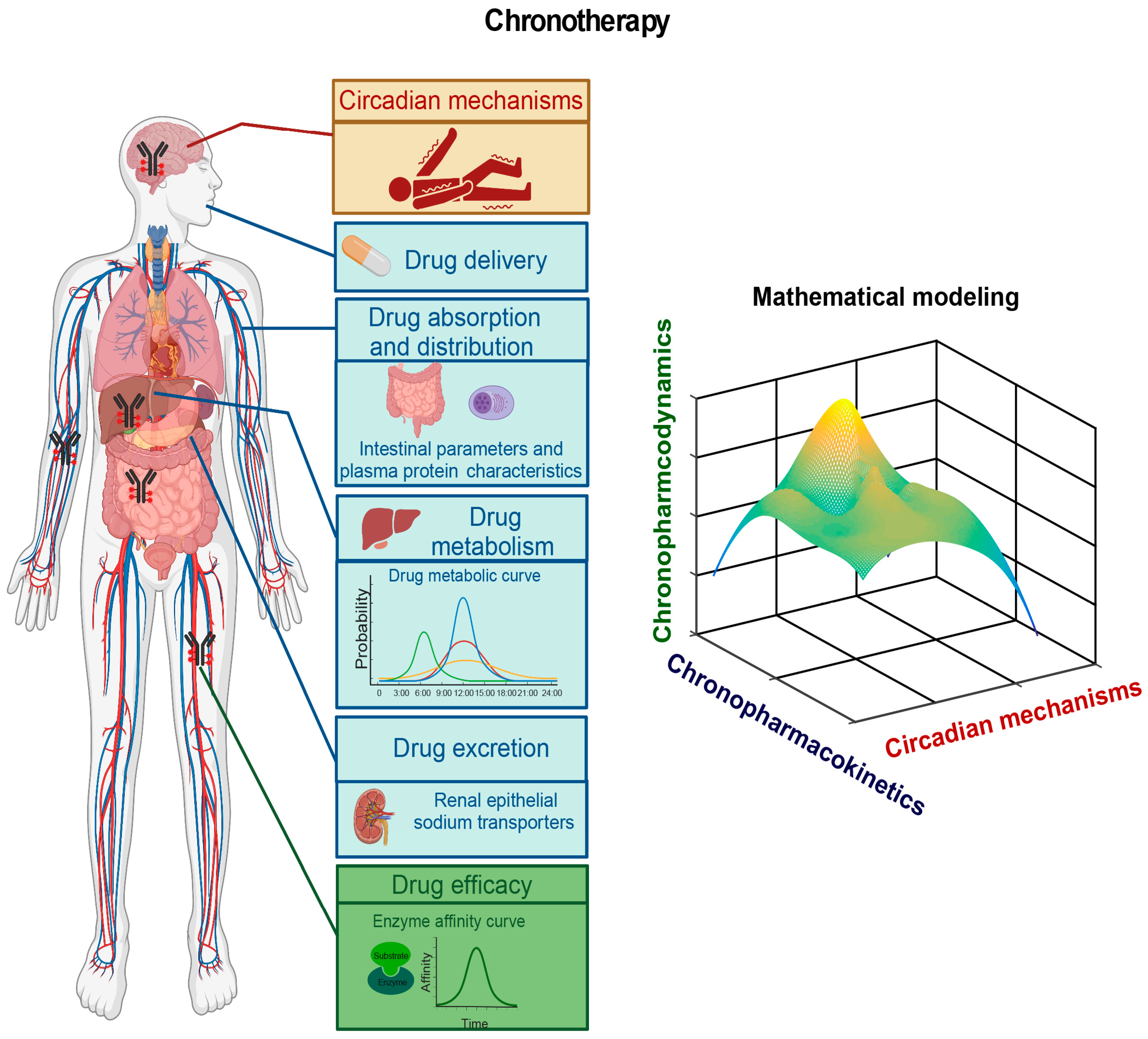
Figure 2. A chronomodulated strategy for epilepsy chronotherapy. Three lines of experiments are required for developing chronomodulated strategy-based epilepsy chronotherapy: (1) to investigate circadian mechanisms underlying time-of-day-specific dynamics of rhythmic epilepsies (red), (2) to investigate chronopharmacokinetics of specific AEDs (blue), and (3) to investigate chronopharmacodynamics of specific AEDs (green). The findings from these three lines of experiments should help develop chronomodulated strategy-based chronotherapy for specific rhythmic epilepsy. Mathematical/computational modeling is also needed to help select an optimal chronotherapy plan.
6.2. Pharmacokinetic and Pharmacodynamic Studies of AEDs
The circadian clock has been known to contribute to pharmacokinetics and pharmacodynamics
[88]. Chronopharmacokinetics investigates how the circadian clock regulates drug absorption, distribution, metabolism, and excretion (ADME), each of which plays a critical role in regulating drug levels in the body
[89][90]. In particular, peripheral molecular clocks in several vital organs, such as the intestine, liver, and drug target tissues, play a direct role in regulating blood drug levels. The absorption of oral drugs depends on the intestinal tract’s physiological parameters
[91]. Considerable evidence has shown the importance of circadian clocks in intestinal physiology
[92].
Furthermore, chronopharmacodynamics focuses on how the circadian clock regulates the factors that affect drug efficacy
[88]. The circadian clock acts through the genes encoding drug targets, transporters, and enzymes, as well as those involved in intracellular signaling pathways, to exert effects on drug efficacy
[93]. Together, chronopharmacokinetic and chronopharmacodynamic studies of an AED should help develop a chronomodulated schedule for time-of-day-specific drug delivery to maximize its efficacy and minimize its toxicities/side effects (
Figure 2).
6.3. Epileptic Chronotherapy
In a study with a small cohort of 17 children with nocturnal or early-morning seizures, instead of conventional administration of equal doses of AEDs in the morning and evening each day, two times the morning AED dose was delivered in the evening with the equivalent total dosage. After the 5-month differential dosing treatment, 64.7% (11/17) of patients became seizure-free, and 88.2% (15/17) experienced a ≥50% reduction in seizures
[19]. AED therapy with CBZ treatment was shown to significantly reduce urinary melatonin metabolite levels of epileptic patients during 06:00–14:00 and 22:00–06:00
[71]. Further, a 5–10 mg evening melatonin delivery can effectively reduce the frequency of epileptic attacks
[94].