Mammalian cells generally require molecular oxygen to produce ATP as an energy source through oxidative phosphorylation (OXPHOS) in mitochondria. However, certain types of cells, such as cancer cells and macrophages, are constitutively dependent on ATP production through an alternate metabolic pathway called glycolysis, and this phenomenon is known as the Warburg effect
. Hypoxia-inducible factors (HIFs), especially HIF-1α, are transcription factors that have roles in immune response, angiogenesis, cell growth, metabolism, and cell death
. Owing to the multiple roles of HIF-1α, its activity is associated with various diseases (e.g., inflammatory and metabolic diseases and cancer proliferation and metastasis)
. Therefore, the proper regulation of HIF-1α is essential for maintaining healthy metabolism and homeostasis.
As a key factor associated with the adaptation of cells to low-oxygen environments, HIFs are known to play crucial roles as transcription factors
[8][9]. HIF proteins include three alpha subunits (HIF-1α, HIF-2α, and HIF-3α) and one beta subunit (HIF-1β); they are heterodimers composed of one of the three alpha subunits and HIF-1β. HIF alpha subunits are stably expressed under hypoxic conditions, whereas HIF-1β is stably expressed independent of oxygen
[10]. All HIF proteins share a basic domain and a dimerization domain within the N-terminal. However, alpha subunits but not beta subunits possess an N-terminal transactivation domain (NAD) in the C-terminal, and only HIF-1α and HIF-2α have the C-terminal transactivation domain (CAD)
[11][12]. These structural differences among the four types of HIF subunits lead to differences in protein stability and transcriptional activity regulation. The HIF subunits also show variations in their expression locus and target genes. While HIF-1α is ubiquitously expressed in human tissues and upregulates the expression of glycolysis-related genes, HIF-2α is expressed in specific tissues, such as tumor vascular cells and macrophages, and promotes erythropoietin and iron metabolism
[13][14][15]. HIF-3α has an important role in adipogenesis, and its gene expression is regulated by HIF-2α activity
[16]. The stability of all alpha subunits is conditionally regulated by oxygen concentration.
3. Mechanisms of Mint3-Mediated HIF-1 Activation
3.1. Mint3 Indirectly Upregulates HIF-1α Activity through Its Interaction with FIH-1
Mint3, also known as amyloid-beta A4 precursor protein-binding family A member 3, is a novel protein that positively regulates HIF-1α
[17]. The Mint family consists of three isoforms: Mint1, Mint2, and Mint3 (also known as APBA1, APBA2, and APBA3; X11, X11-like, and X11-like2; and X11α, β, and γ, respectively). Mint1 and Mint2 bind with Munc-18-1, which is involved in neural homeostasis
[18]. Mint3 shows ubiquitous expression in various tissues, with the lowest level in the testis, according to Okamoto and Sudhof
[19]. Its intracellular localization is mainly at the Golgi apparatus where it interacts with furin
[20]. Mint1-3 were originally identified as neural proteins that interact with the YENPTY motif within amyloid precursor proteins (APPs), which are conserved in the cytoplasmic region of APP and modulate their activities
[19][21][22]. All three Mint isoforms share highly conserved structures in their C-terminal region, which comprise one phosphotyrosine-binding (PTB) domain followed by tandem PDZ domains (PDZa and PDZb). Through these two domains, Mint3 functions as an adaptor protein. With its PTB domain, Mint3 binds to proteins such as furin, N-terminal EF-hand calcium binding protein 3 (NECAB3), and Rab6
[19][20][23][24].
However, only Mint1 and Mint2 retain the N-terminal region, which contains Munc-18-1 interacting domain; because Mint-3 lacks the domain, it is unable to interact with Munc-18-1
[19] (
Figure 1). Additionally, a recent structural analysis of Mint3 revealed that its N-terminal region is intrinsically disordered
[25]. Thus, the sequences of the N-terminal region of Mint3 differ substantially from those of the other Mint proteins, and, as a result, Mint3 has divergent binding partners at its N-terminal region.
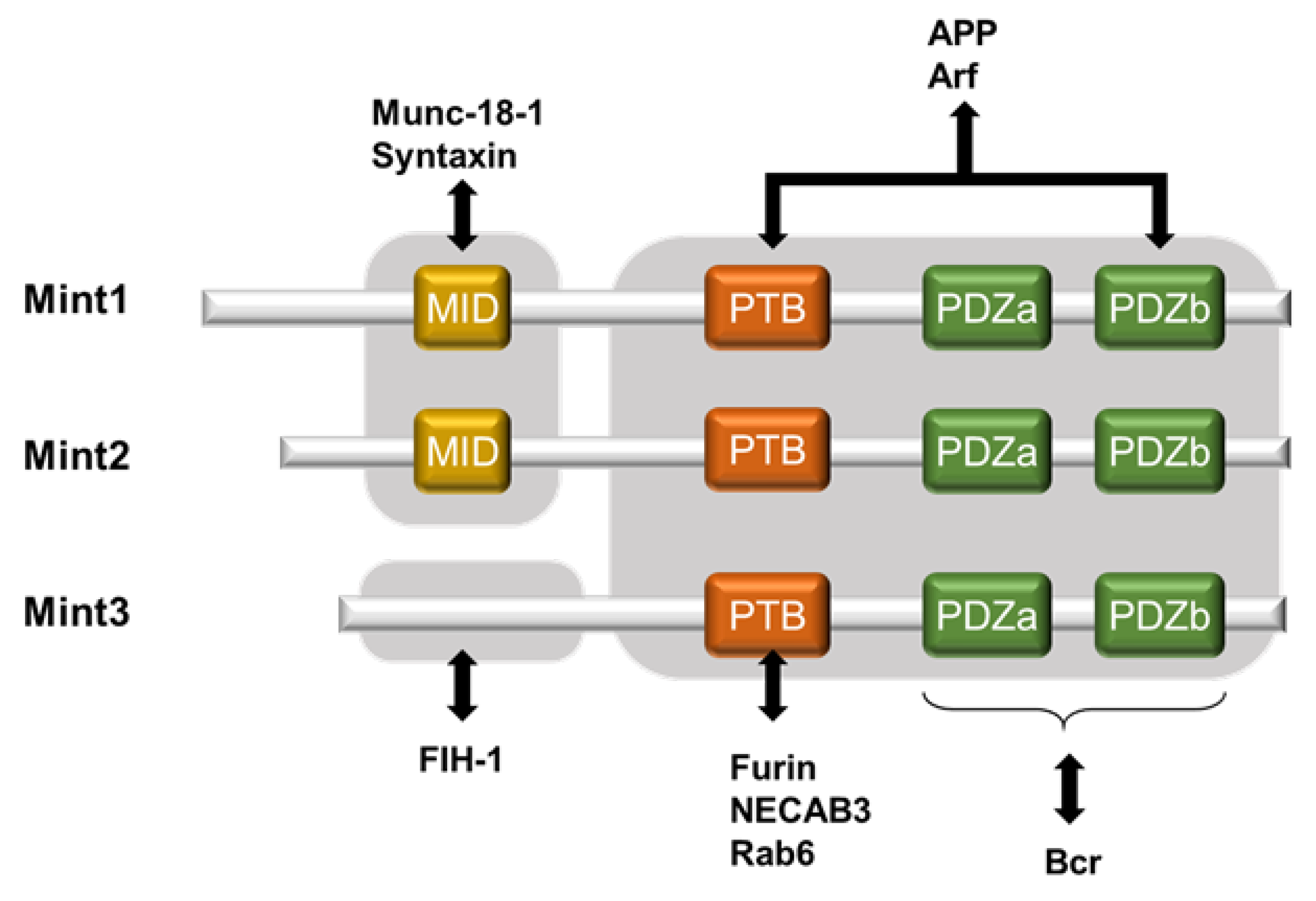
Figure 1. The schematic structures of Mint proteins with their binding partners. Mint family comprises well-conserved C-terminal region with one phosphotyrosine-binding domain (PTB) and tandem PDZ domains (PDZa and PDZ b); differential N-terminal region with Munc-18-1 interacting site (MID) only for Mint 1 and 2; FIH-1 binding site for Mint3. FIH-1—Factor inhibiting HIF-1, NECAB3—N-terminal EF-hand calcium binding protein 3, APP—amyloid precursor protein, and Arf—ADP-ribosylation factor (Arf)-GTPase.
3.2. Factors That Support Mint3-Mediated HIF-1α Activation
Although Mint3 is ubiquitously expressed, its effect on HIF-1α regulation is limited to specific regions where MT1-MMP is expressed simultaneously, such as fibroblasts, macrophages, and cancer cells. MT1-MMP, also known as MMP14, is a member of the MMP family. The MMP family comprises 23 members, most of which are composed of well-conserved structures. The extracellular domains include the pro-domain, catalytic (CAT) domain, which binds with zinc ions, and hemopexin (HPX) domain; in addition, a transmembrane region and an adjacent cytoplasmic region are present
[26]. Among the 23 MMPs, 6 are recognized as membrane-anchored MMPs, which are further classified into two types as follows: 1) MT1-MMP (MMP14), MT2-MMP (MMP15), MT3-MMP (MMP16), and MT5 (MMP-24) are transmembrane types, which conserve the cytoplasmic region, and 2) MT4-MMP (MMP-17) and MT6-MMP (MMP-25) are glycosylphosphatidylinositol-anchored types, which lack the cytoplasmic region
[26][27]. MT1-MMP is initially expressed in an inactive state (pro-MMP); however, it switches to the active form upon proteolytic processing in the pro-domain by furin
[28]. MT1-MMP is the first transmembrane MMP type to be associated with the upregulation of invasion and the metastasis of cancer cells
[29]. This is somewhat convincing because the most important substrates of MT1-MMP are the extracellular matrix (ECM) molecules, especially type-1 collagen, with MT1-MMP catalyzing various extracellular proteins, including secreted proteins (e.g., growth factors, cytokines/chemokines, and collagens) and cell surface proteins (e.g., adhesion molecules and transmembrane receptors)
[30][31].
Apart from the functions of MT1-MMP as a proteinase, it also plays an important role in HIF-1α activation by supporting the Mint3–FIH-1 interaction. Twenty amino acids within the cytoplasmic tail (CPT) of MT1-MMP enable FIH-1 to have direct contact with MT1-MMP, resulting in the inhibition of FIH-1′s suppression of HIF-1α activity
[32][33].
4. Mint3 Mediates Inflammatory Responses
Macrophages are the key players in innate immunity and rely on constant HIF-1α-mediated glycolysis regardless of oxygen conditions
[4][34], which is dependent on Mint3 activity. Upon stimulation or infection by various pathogens, macrophages are involved in producing cytokines and chemokines, growth factors, and reactive oxygen species (ROS) during inflammation to protect the host. However, the overactivation of the immune system can rather be toxic due to septic shock
[35]. Stimulation by lipopolysaccharides (LPS), which are a toxic component of the outer membrane of Gram-negative bacteria, causes septic shock. This toxicity is a consequence of a cytokine storm mediated by the glycolysis-dependent secretion of cytokines associated with increased motility and invasiveness of macrophages, which involve the Mint3–FIH-1–HIF-1α axis
[36]. Macrophages gain “lethal force” by Mint3 expression under LPS-driven immune reaction.
In an immune response to influenza virus (IFV), Mint3 depletion efficiently improves influenza pneumonia by alleviating the production of cytokines and chemokines in macrophages (but not in dendritic cells) through two mechanisms: the upregulation of Adenosine 5′-monophosphate (AMP)-activated protein kinase (AMPK) α activity and the stabilization of IκB
[37]. AMPK is activated through its phosphorylation on Adenosine 5’-triphosphate (ATP) starvation
[38] and downregulates nuclear factor kappa B (NF-κB) activity
[39]. The depletion of Mint3 downregulates glycolysis-mediated ATP production and activates AMPK and the subsequent inhibition of NF-κB. Concurrently, Mint3 deficiency leads to the glycolysis-independent stabilization of IκB, which causes the simultaneous inhibition of NF-κB.
Pyroptosis is a mechanism of programmed cell death that is associated with innate immunity and occurs when cells encounter intracellular bacterial infection. It was initially pointed out by Friedlander and was named by D’Souza to distinguish it from apoptosis
[40][41]. Canonical pyroptosis signals involve the formation of inflammasomes, which mostly comprise Nod-like receptors (NLRs)/absent in melanoma 2 (AIM2); apoptosis-associated speck-like protein (ASC) as an adaptor protein; and the inflammatory caspase-1, whereas caspase-11/4/5 could serve as an inflammatory caspase in non-canonical pyroptosis. Inflammasomes process the pyroptosis-executing protein gasdermin D (GSDMD) and interleukin (IL)-1β/IL-18.
Listeria monocytogenes (LM) is a common foodborne pathogen that causes zoonotic diseases in Western countries by infecting various types of cells. Upon innate infection by LM, the host operates a protective mechanism by clearing out the bacteria through the production of ROS and nitric oxide (NO) induced by the activation of pyroptosis
[42][43][44][45].
5. Role of Mint3 in Cancer Progression
The effects of Mint3 on the innate immune system may be applicable in cancers because Mint3 has the potential to increase cancer malignancy and metastatic features through its expression in inflammatory monocytes (IMs), which are defined with the following markers: Gr-1/Ly6C+, CD11b/CD115+ [46]. In addition, a relationship between Mint3 activity in cancer cells and cancer-associated fibroblasts (CAFs) has been previously reported [47][48][49]. Because Mint3 has key roles in enhancing cancer progression and metastasis by upregulating HIF-1α, which is also related to the tumor microenvironments, it could be an attractive target for cancer treatment, as tumor-microenvironments-related factors are gaining more attraction, which is described as one of the important hallmarks of cancers [50].
5.1. Impact of Mint3 Activity in Cancer Cells
Solid tumors in human bodies are often exposed to low-oxygen environments due to the disadvantage in physical distance from the blood vessels and obtaining sufficient oxygen. In order to adapt to such circumstances, cancer cells tend to retain high HIF-1α activities and gain malignancies. Although the depletion of Mint3 in the host of xenograft models has no effects on tumor progression, its depletion in cancer cells results in HIF-1α suppression, which is associated with downregulated glycolysis, angiogenesis, and anti-proliferative effects. These Mint3-related features are commonly seen in the xenografts of various types of cancer cells (e.g., breast cancer, MDA-MB-231; fibrosarcoma, HT-1080; epidermoid carcinoma, A431; non-small cell lung cancer, A549; and urothelial carcinoma, RT-112)
[47][48][49][51].
5.2. Metastatic Ability of Cancer Cells Achieved through Mint3 Expression in IM
Using the PyMT breast cancer mouse model, which develops palpable breast cancers that metastasize to the lung, Qian et al. reported that IMs, which are characterized by Gr-1/Ly6C
+, CD11b/CD115
+ markers, play a key role in the lung metastasis of breast cancer in a C–C motif chemokine ligand 2 (CCL2)-dependent manner
[52]. Other studies using xenograft mouse models of various types of cancers have shown that Mint3 plays an important role in this mechanism of metastasis
[46][53]. Cancer cells and the surrounding stromal cells are the sources of CCL2, and they increase the number of IMs in the peripheral blood. Both individual depletion of Mint3 or HIF-1α in IMs leads to the decreased production of vascular endothelial growth factor (VEGF) to comparable levels. These effects of Mint3 or HIF-1α depletion on VEGF expression are consistent with the fact that VEGF is one of the target genes of HIF-1α
[46][54].
5.3. Supportive Effect of Mint3 Expression in CAFs on Cancer Progression
CAFs are one of the factors that have a crucial role in promoting cancer malignancy through ECM remodeling, immune crosstalk, and metabolic effects
[55]. These features of CAFs are achieved by functioning as a source of various secretory proteins, such as growth factors, cytokines, and exosomes, whereas the adherent molecules in CAFs could also increase tumor malignancy in a Mint3-dependent mechanism. Mint3 is also involved in the proliferation of cancer cells by regulating the expression of L1 cell adhesion molecule (L1CAM) in fibroblasts. L1CAM is a cell adhesion molecule that acts as a binding partner of heterodimeric integrins, such as α5β1, αvβ3, αIIbβ3, and αvβ5
[56][57], and triggers downstream signaling pathways, such as the MAPK and PI3K/Akt pathways
[56][58]. Fibroblasts express L1CAM in a Mint3-dependent manner, which enables CAFs to promote direct contact with cancer cells expressing integrin α5β1. Therefore, Mint3 also plays a role in enhancing the proliferation of cancer cells through CAFs
[47].
6. Therapeutic Efficacy of Targeting Mint3-Related Environments
In some cases, the activity of HIF-1α is toxic in terms of its ability to promote inflammatory diseases or cancer malignancies. Thus, HIF-1α has long been regarded as a beneficial therapeutic target. Many efforts have been made to develop HIF-1α inhibitors, but none have been successful, although some clinical trials are still underway. This is probably due to the ubiquitous expression of HIF-1α, which influences various factors. The first HIF-2α inhibitor, belzutifan (Welireg), was clinically approved for patients with VHL diseases and some types of cancers in 2021. Naphthofluorescein (Naph) was first identified as a potent Mint3 inhibitor. It effectively disrupts the protein interaction between Mint3 and FIH-1, leading to the suppression of HIF-1α activity with reduced expression of HIF-1α-targeting genes, glycolysis, and ATP production
[51]. The inhibitory effect of Naph on Mint3 in inflammatory diseases effectively diminishes cytokine synthesis; upon LPS stimulation, this is well reflected in the increased survival of mice with LPS-induced inflammatory diseases. Moreover, Naph retains its inhibitory potential against chemotaxis of IMs toward CCL2–expressing cancer cells and the surrounding stromal cells, thereby reducing the expression of E-selectin in metastatic lung cancers without marked adverse effects. Notably, the pharmacological effect of Naph on metastasis is not affected in Mint3-deficient mice, which implies that Naph can suppress metastasis specifically by inhibiting Mint3
[51].