2. Au NPs-Based Colorimetric Assay of Metal Ions via Anti-Aggregation
In regard to the anti-aggregation-enabled Au NPs-based colorimetric metal ions detection, quantification of Hg
2+ has attracted much attention by numerous researchers
[47][48][49][50][51][52][53][54][55][56][57]. Li and co-workers described the use of label-free citrate–capped Au NPs (CI-Au NPs; particle size = 13 nm; (nm = nanometer)) as a colorimetric probe toward mercury ions detection via the anti-aggregation strategy
[47]. In this work, the 4,4′-dipyridyl (DPy; 5.2 µM (µM = micromole)) acted as an aggregation-inducing agent of Au NPs that can be released from the NPs’ surface because of its exceptional coordination ability toward Hg
2+. When the 4,4′-dipyridyl induces Au NPs aggregation, the color changes from red to blue. However, in the presence of Hg
2+, it changes back to its original red color due to anti-aggregation, as represented in
Figure 2A. None of the metal ions, except Hg
2+, displays colorimetric reversibility as seen in
Figure 2B. The best result was obtained at pH 7 (buffer: 10 mM Tris(hydroxymethyl)aminomethane hydrochloride (Tris-HCl)) after 30 min incubation (min = minutes). Transmission electron microscopy (TEM) studies clearly attested the anti-aggregation of Au NPs. Surface plasmon resonance (SPR at A
520/A
620) studies revealed the linearity to be between 0.04 and 1.1 µM, with an estimated limit of detection (LOD) of 15 nM (nM = nanomole). This work was also demonstrated with tap water and spring water samples (with 100-fold excess interference ions) investigations; therefore, it can be utilized for Hg
2+ quantification in real time.
Figure 2. (
A) Schematic representation of anti-aggregation of Au NPs for the detection of Hg
2+; (
B) absorbance ratio of A
520/A
620 and photographic images (inset) of solutions containing Au NPs, DPy (4,4′-Dipyridine; 2.5 µM), and various metal ions (each 100 µM, except Hg
2+ 1.0 µM) ((
A,
B) are reproduced with the permission from
[47]); (
C) schematic illustration of the determination of Hg
2+ by PDDA-Au NPs in the presence of Cys; (
D) the SPR band shift relative to Cys-Au NPs (∆λ) and photographic image of Au NPs solutions containing 0.7 µg/mL Cys, 1.0 × 10
−4 M EDTA-Na
2, and 1.0 × 10
−5 M various metal ions. ((
C,
D) are reproduced with the permission from
[48]).
Following a similar approach, Ding et al. described anti-aggregation-mediated colorimetric detection of Hg
2+ by dislocating the aggregation-inducing agent cysteine (Cys; 7 µg/mL) at 0.1 M NaCl
[48]. In the study, positively charged Au NPs were synthesized using poly(diallyldimethylammonium) chloride (PDDA) to afford PDDA-Au NPs (particle size = 12.2 nm). The PDDA acted as a reducer and stabilizer at the same time. Adding the Cys into PDDA-Au NPs led to a color change from ruby red to royal purple via aggregation. In the early stage, the aggregation was negligible due to the strong electrostatic repulsion between PDDA-Au NPs and Cys. However, after adding 0.1 M of NaCl, the electrostatic repulsion became weaker, and the Cys-Au NPs aggregates were formed. A pH value of 5 (Buffer: acetic acid–sodium acetate (HAc-NaAc)) was selected to enhance the strong Au-S binding (via zwitterionic Cys form) in the Cys-Au NPs aggregates. Owing to the strong interaction between Cys and Hg
2+, it was more favorable for the Hg-Cys complex formation; therefore, it prevented the Cys-Au NPs aggregates and reversed the color back to ruby red. This anti-aggregation behavior was demonstrated by TEM and SPR (at A
520/A
610) studies. Among other metallic species, Pb
2+ and Cu
2+ also displayed a certain degree of anti-aggregation by forming a complex with Cys, which can be avoided by using the masking agent EDTA-Na
2 (ethylenediaminetetraacetic acid disodium salt).
Figure 2C,D demonstrate the anti-aggregation of the Au NPs-Cys system in the presence of Hg
2+. The Hg
2+ shows the linear range to be between 0.05 and 10 µM with an LOD of 25 nM. This work was demonstrated in drinking water samples (>100% recovery), but more interrogations are required to further understand the underlying mechanism.
Aggregation of citrate-capped (CI) Au NPs (particle size = 13 nm; conc. = 15 nM (conc. = concentration)) was induced by thymine via citrate replacement from the NPs’ surface. When Hg
2+ was present, it interacted with thymine to afford anti-aggregation-enabled reversibility of the original SPR and color
[49]. In the presence of thymine (0.2 mM), the color of CI-Au NPs turned to blue. When Hg
2+ was added, a “thymine-Hg
2+-thymine” motif was formed, which released the thymine from the Au NPs’ surface and reversed the color back to the original red through the anti-aggregation (see the TEM and SPR (at A
520/A
670) interrogations). Optimum pH condition and incubation time were established as pH 8 (buffer: 1 mM Tris-HCl) and 30 min. None of the ions, except the Hg
2+, induced anti-aggregation and exhibited significant recoveries (88–112.5%) in tap water samples. This report can be regarded as a novel work in terms of its uncomplicated procedure, linearity (2–12 µM), LOD (2 nM), and real-time applicability. Subsequently, O-phenylenediamine (OPD; 3.1 µM) induced CI-Au NPs aggregations (particle size = 7–11 nm) and displayed a color change from red to blue
[50]. By adding Hg
2+ to the OPD-Au NPs complex system, the anti-aggregation was initiated via OPD-Hg
2+ complexes formation (after 20 min incubation time), and the color was changed back from blue to red. The effective coordination between Hg
2+ and OPD is directed by two amine (-NH
2) groups present in OPD at an operative pH value of 6.5. As seen in
Figure 3A,B, none of the metal ions, except Hg
2+, shows high selectivity via anti-aggregation. This was also confirmed by TEM, SPR (at A
520/A
680), and density functional theory (DFT) investigations as well. The linear regression of Hg
2+ was between 0.01 and 2 µM, with an LOD of 5 nM. This work was applied to tap water and lake water samples interrogations, which showed recoveries of between 70 and 120%. In terms of its high colorimetric selectivity, linear range, LOD, theoretical support, and real sample studies, this report can be regarded as a unique work in Hg
2+ quantification.
Figure 3. Selectivity of the Au NPs-based detection system (containing 3.1 µM of OPD) for Hg
2+ compared with other ions. (
A) Photograph of the detection systems incubated with Hg
2+ (2.0 µM) or other ions (20 µM); (
B) A
520/A
680 (ratio of the absorbance value at 520 nm to that at 680 nm in the UV–vis spectra) of the detection systems incubated with Hg
2+ (2.0 µM) or other ions (20 µM) (mean ± SD,
n = 3) ((
A,
B) are reproduced with the permission from
[50]).
The CI-Au NPs were engaged in anti-aggregation-enabled colorimetric detection of Hg
2+ as described by the following: The L-penicillamine (Pen; optimum concentration fixed as 3.5 µM) induced aggregation of CI-Au NPs (particle size = 20 nm; conc. = 2.85 nM) as evident from the emerging of a new absorbance peak at 750 nm. The absorption peak was blue-shifted to 525 nm due to anti-aggregation and effective binding of penicillamine to Hg
2+ (via Pen-Hg
2+-Pen complex) when Hg
2+ was added
[51]. Both aggregation and anti-aggregation were attested by TEM studies. Detection of Hg
2+ showed the linearity as being between 50 and 400 nM (at A
525/A
750), with an LOD of 25 nM. This work was supported by spiked tap water investigations, but the appropriate pH for this sensor was predicted to be acidic pH 2.4 (buffer: 5 mM citric acid–sodium citrate), thereby requiring more clarifications on its reliability. Zhou et al. utilized the 4-mercaptophenylboronic acid (MPBA; 10 µM) as an aggregation-inducing agent with CI-Au NPs (particle size = 15 nm) for colorimetric detection of Hg
2+ by means of anti-aggregation
[52]. The citrate attached on the NP surface was replaced by MPBA (via Au-S bond) and led to aggregation, thereby changing the color from red to blue.
When Hg2+ is present, the MPBA coordinates with Hg2+ via strong Hg-S interactions to initiate the anti-aggregation accompanied with a color change from blue to red as demonstrated in Figure 4A. The best result was attained at pH 4 (buffer: 10 mM citric acid–sodium citrate) after 20 min incubation.
Figure 4. (
A) Schematic presentation of the Au NPs colorimetric mechanism for Hg
2+ detection; (
B) photographic image; (
C) UV–vis absorbance spectra and (
D) linear plot of the A
520/A
690 vs. Hg
2+ concentration (c) of the MPBA (10 µM)-Au NPs solution (pH 4.0) containing various concentrations of Hg
2+: (1) 0, (2) 0.01, (3) 0.05, (4) 0.1, (5) 0.2, (6) 0.8, (7) 1.2, (8) 2, (9) 3, (10) 4, (11) 5, (12) 6, (13) 8, and (14) 10 µM. ((
A–
D) are reproduced with the permission from
[52]).
Detection of Hg
2+ shows colorimetric response and linear regression as being between 0.01 and 5 µM (at A
520/A
690), with an LOD of 8 nM, as seen in
Figure 4C,D. TEM studies confirmed the anti-aggregation in the Hg
2+ detection, and none of the other metal ions displayed the interfering effect. Moreover, spiked lake water interrogations showed recoveries of between 96.3 and 110%, thereby approving its real-time applicability. In a similar fashion, free thiol (-SH) containing aggregation-inducing agents, such as N-Acetyl-L-cysteine (NAC; 10 µM; 30 min incubation time; pH 7 (buffer: phosphate buffer saline (PBS) prepared from NaH
2PO
4 and Na
2HPO
4)) and 2-Mercapto-benzothiazole (MBT; 2.2 µM; 5 min incubation time; pH 5.8 (buffer: PBS)), were used in discrimination of Hg
2+ [53][54]. Both agents induced aggregation of CI-Au NPs (particle size = 15 nm (conc. = 15 nM) and 10–20 nm, respectively) and observed a color change from red to blue, which was then disrupted by Hg
2+-enabled anti-aggregation with a reversed color change from blue to red. The dimeric complexes (NAC-Hg-NAC and MBT-Hg-MBT) were formed with those reactive agents because of the high binding tendency of Hg to S. The linear regression of Hg
2+ was reported to have values between 0.02 and 1 µM (at A
520/A
620) and 0.05–1 µM (at A
700/A
520), with calculated LODs of 9.9 nM and 6 nM, respectively. Both reports were well authorized by TEM and recovery studies in real water and milk samples analysis; therefore, they can be regarded as notable reports in anti-aggregation-mediated Hg
2+ quantification.
Rajeshwari and co-workers proposed a tween 20-modified gold nanorods (Au NRs; average length = 45.11 ± 0.64 nm and diameter = 17.95 ± 0.38 nm)-based detection of Hg
2+ via the anti-aggregation strategy
[55]. Herein, dithiothreitol (DTT; 1.5 µM) firstly induced aggregation of NRs. When Hg
2+ was added, the strong binding affinity of DTT to Hg
2+ turned the aggregations into dispersed NRs. The linear regression of Hg
2+ was between 1 and 100 pM (SPR at 679 nm; pH 7.4 (buffer: Tris-HCl); pM = picomole), with an LOD of 0.42 pM. This work also conducted studies in stimulated body fluid recovery, but they lacked colorimetric responses. Sun et al. utilized the hexadecyl trimethyl ammonium bromide (CTAB; 0.44 µM) to induce aggregation of CI-Au NPs (particle size = 13 nm; conc. = 8.7 nM), which was dis-aggregated by Hg
2+ via anti-aggregation with a corresponding color change from blue to red
[56]. The best pH and response time were determined to be pH 7 (buffer: Tris-HCl) and 30 min. In the beginning, aggregation of NPs took place due to strong electrostatic attraction between CI-Au NPs and CTAB, with a respective color change from red to blue. In the presence of Hg
2+, Au NPs tend to form a Hg-Au alloy, which hinders the electrostatic attraction and inhibits aggregation caused by CTAB, as shown in
Figure 5A. The linear range of Hg
2+ was between 0 and 0.68 µM (at A
650/A
520), with an LOD of 11.9 nM. The anti-aggregation was confirmed and attested by TEM and DLS (DLS = dynamic light scattering) investigations and by tap water recovery (>90%; RSD ≤ 4% (RSD = relative standard deviation)). Therefore, it can be regarded as an important probe toward Hg
2+ quantitation. Thereafter, 3, 5-Dimethyl-1-thiocarboxamidepyrazole (Pzl; 1.5 µM)-induced aggregation of CI-Au NPs (particle size = 14 nm; conc. = 15 nM) was utilized for the colorimetric detection of Hg
2+ [57]. In the presence of Pzl, the color of the CI-Au NPs changed from red to blue via aggregation, which was reversed (from blue to red) in the presence of Hg
2+ by means of anti-aggregation. The reason of the color reverse to the original (as shown in
Figure 5B) is that the Pzl goes through coordination with Hg
2+ to form a Pzl-Hg-Pzl complex due to the presence of the functional unit -C = S. None of the ions, except Hg
2+, displayed interfering selectivity. The optimum response was found at pH 5.6–8 (buffer: PBS) after 3–4 min incubation. The anti-aggregation mechanism and dimeric mercury complex of Pzl were clarified and confirmed by TEM studies and DFT optimization, respectively. The linearity of Hg
2+ was between 0.01 and 1.5 µM (at A
680/A
520), with an LOD of 7.7 nM, and was attested by recovery studies in tap water and lake water (lies between 94 and 105%). Based on the TEM, DLS, and real samples analysis, this report can be regarded as a well accomplished work.
Figure 5. (
A) The sensing mechanism of the colorimetric sensor based on anti-aggregation of Au NPs for Hg
2+ detection (reproduced with the permission from
[56]) and (
B) schematic representation of the 3, 5-dimethyl-1-thiocarboxamidepyrazole (Pzl)-derived colorimetric detection mechanism for Hg
2+ (reproduced with the permission from
[57]).
Other than the Hg
2+ detection, anti-aggregation-mediated discrimination of Ag
+ was also demonstrated with CI-Au NPs, as described next. Mao et al. described CI-Au NPs’ (particle size = 13 nm) anti-aggregation-tuned selective detection of Ag
+ [58]. Herein, the thiamazole (2 µM) was used as an aggregation-inducing agent to produce an Au NPs color change from red to blue. The aggregation was then inhibited in the presence of Ag
+. Due to Ag
+-enhanced oxidization of sulfhydryl groups (-SH), anti-aggregation takes place to reverse the color from blue to red, as illustrated in
Figure 6A. TEM study confirmed the involvement of the anti-aggregation strategy with the optimum pH value and response time fixed at 7.5 (buffer: Britton Robison (BR) buffer solution made up from H
3PO
4, H
3BO
3, and HAc (0.04 M)) and 15 min. The dynamic linear range of Ag
+ was between 0.1 nM and 90 μM (at A
520/A
660), with an LOD of 0.042 nM. Note that there was no interfering effect in the presence of other ions, and real water (tap and river) samples displayed recoveries of >98%. This is a nice work (toward Ag
+ quantification) in terms of its performance in the linear range, LODs, and real-time applicability.
Figure 6. (
A) Schematic representation of the analytical process for Ag
+ detection (reproduced with the permission from
[58]); (
B) schematic representation of the Au NPs-based colorimetric assay for silver; (
C) color changes with increasing concentration of Ag
+ from 0 to 9 µM; (
D) SPR absorption change of Au NPs in tris solution with Ag
+ concentration varied from 0 to 9 µM; (
E) the linear response of the system to Ag
+ ion concentration. (The concentration of tris solution was 9 mM, and the pH was fixed at 7.5. Au NPs’ concentration was 1.2 nM.) ((
B–
E) are reproduced with the permission from
[59]).
Label-free Au NPs (particle size = 12 nm; synthesized via NaBH
4 reduction; SPR at 512 nm; conc. = 20 nM) composited with ascorbic acid (AA) were engaged in Ag
+ detection without employing any aggregation agent
[60]. The AA-tuned reduction of Ag
+ resulted in Ag
0 on Au NPs’ surface and formed an Ag–Au alloy. The linearity of Ag
+ was between 2 and 28 µM (SPR at 385 nm), with a calculated LOD of 0.85 µM. Even though this work was demonstrated in drinking water samples, it cannot be categorized as an anti-aggregation strategy-based sensor. Colorimetric detection of Ag
+ based on anti-aggregation of CI-Au NPs (particle size = 20 nm; conc. = 1.2 nM) was proposed by Safavi and co-workers
[59], wherein tris(hydroxymethyl) aminomethane (tris at 9 mM; pH 7.5) was used as the aggregation-inducing agent to deliver a color change from red to blue, with a new SPR peak occurring at 650 nm. The anti-aggregation-enabled colorimetric response is observed with a color change from blue to red (within 20 min) due to the strong interaction between tris and Ag
+, as represented in
Figure 6B. The Ag
+-mediated anti-aggregation was well established from TEM, SPR, and interference studies. The color change (from blue to red), SPR changes, linear range (1–9 µM; at A
520/A
650), and LOD (0.42 µM) calculations are shown in
Figure 6C–E. This work was demonstrated by wastewater and river water investigations with a recovery >96%; therefore, it can be regarded as a nice innovation.
Gao et al. described the anti-aggregation-facilitated detection of Cu
2+ using CI-Au NPs (particle size = 18 nm; conc. = 3.89 nM) with glutathione (GSH; 5 µM) as the aggregation-inducing agent
[61]. The free thiol (-SH) of GSH replaced the citrate from the Au NPs’ surface to induce the aggregation in a short time and produced a color change from red to blue with a corresponding SPR shift from 520 nm to 650 nm. In the presence of Cu
2+, the GSH was oxidized to form GSSG, and the anti-aggregation took place to reverse the color from blue to red, with a shifting of the SPR peak from 650 nm to 520 nm. The best result was obtained at pH 3.4 (buffer: 4 mM BR) after 15 min incubation. It displayed a linear regression of between 20 and 500 nM (at A
650/A
520), with an LOD of 20 nM with Cu
2+, and was also demonstrated by tap water recovery (>96%; RSD ≤ 3%). Moreover, colorimetric reversibility of Cu
2+ with S
2− was also discussed in this report. Therefore, based on TEM, colorimetric/SPR responses, interference, kinetic, temperature, and reversibility investigations, this report can be attested as an exceptional work in Cu
2+ detection. Thereafter, D-penicillamine (D-PC; 30 µM)-enabled aggregation of CI-Au NPs (particle size = 13 nm; conc. = 15 nM) was demonstrated in detecting Cu
2+ via the anti-aggregation strategy
[62]. The D-PC induced the aggregation via providing its free -SH unit to CI-Au NPs, which resulted in a color change from red to blue. After adding Cu
2+, D-PC coordinated with Cu
2+ to form dimeric complexes (D-PC-Cu-D-PC via the S-Cu-S bond) and enabled anti-aggregation to reverse the color change from blue to red. The best result was found at pH 7 (buffer: 1 M NaCl-10 mM NaOH) in the presence of 25 mM NaCl and after 12 min incubation. The linear range of Cu
2+ was between 0.05 and 1.85 µM (at A
520/A
650), with an LOD of 30 nM. TEM and SPR studies confirmed the anti-aggregation. The real water (tap water, cascade water, and mineral water) interrogations showed recoveries of between 85 and 108% (RSD = 1.43–6.93%). Thus, this work can be adopted in real time detection of Cu
2+; however, careful optimizations on the concentrations of D-PC, NaCl, and pH are required.
In contrast to the aforementioned metal ion sensors in this section, Deng and co-workers described the Schiff base and Lewis acid–base interaction-regulated anti-aggregation for colorimetric detection of Sc
3+ [63], wherein the biomolecule pyridoxal phosphate (PLP; 5 µM; contains free -CHO group) reacted with cysteamine-functionalized Au NPs (Cyst-GNPs; particle size = 17.5 ± 3.4 nm (
n = 100) positively charged with free -NH
3+ at pH 6) to form a neutral Schiff base and induced aggregation to produce a color change from red to blue. The competition between the intense Lewis acid–base interactions of Sc
3+ with PLP and Cyst-GNPs binding to PLP enables the anti-aggregation of Cyst-GNPs to display a reversed color change from blue to red, as shown in
Figure 7. TEM, DLS, and SPR responses, and interference studies confirmed the anti-aggregation-based high selectivity of Sc
3+. The incubation time of all experiments was established as 1 min at pH 6 (buffer: HAc-NaAc). The linear regression of Sc
3+ was between 0.1 and 3 µM (at A
700/A
525), with an estimated LOD of 20 nM. The tap water recoveries of Sc
3+ in this method were between 96.87 and 101.8%, with corresponding RSD values of 0.6–3.6%. This is the only report in the anti-aggregation-mediated colorimetric detection of rare earth Sc
3+; therefore, it can be noted as an exceptional work.
Figure 7. Schematic of PLP-triggered aggregation of Cyst-GNPs for colorimetric detection of Sc
3+ (reproduced with the permission from
[63]).
3. Au NPs-Based Colorimetric Quantification of Anions via Anti-Aggregation
Plaisen and co-workers described the detection of bromide anion (Br
−) via the anti-aggregation strategy
[64] as follows: CI-Au NPs (particle size = 16.43 ± 0.81 nm; conc. = 5.52 nM) aggregation took place in the presence of Cr
3+ ions (optimum conc. = 4.81 µM), which resulted in a color change from red to blue. However, when Br
− was pre-mixed (at pH 6.5 (buffer: 10 mM sodium phosphate (Na
3PO
4)) and 10 min reaction time), the color overturned from blue to red via anti-aggregation. TEM and DLS studies confirmed the involvement of the anti-aggregation strategy. In fact, Br
− tends to affect the citrate capping via self-deposition over the NPs’ surface, which also hinders the binding of citrate to Cr
3+, thereby resulting in the color change. The linear regression of Br
− was found between to be 0.31 and 3.75 µM (at A
519/A
673), with an LOD of 40 nM and a limit of quantification (LOQ) of 0.13 µM. This work was applied in rice sample investigations; thus, it can be regarded as a nice innovation.
Due to the strong binding affinity of Hg
2+ to the iodide anion (I
−), there are experiments and reports in using Hg
2+ as an aggregation-inducing agent to conduct anti-aggregation-based colorimetric detection of I
−. The N-1-(2-mercaptoethyl) thymine-capped Au NPs (particle size = 23 nm; conc. = 1.4 nM), T-rich single-stranded DNA (DNA = deoxyribonucleic acid)-capped Au NPs, (T-rich ssDNA–Au NPs; particle size = 13; conc. = 3 nM), and 2-hydroxyethyldithiocarbamate-capped Au NPs (2heDTC-Au NPs; particle size = 12.92 ± 1.44 nm; conc. = 15 nM) were proposed in Hg
2+-enabled aggregation to engage in I
− detection via the anti-aggregation tactic
[65][66][67]. The mercapto-functionalized thymine and T-rich ssDNA over Au NPs aggregated strongly in the presence of Hg
2+ (300 nM and 2 µM, correspondingly) and induced color changes from red/wine-red to blue/violet-blue
[65][66]. In fact, the aggregation is highly effective due to the strong attraction between thymine and Hg
2+ via the T-Hg
2+-T complex. Upon pre-treating I
− with Hg
2+, the T-Hg
2+-T complexation was interrupted by the HgI
2 formation, thus reversing the colorimetric response from blue/violet-blue to red/wine-red via anti-aggregation. The best pH condition in both reports
[65][66] was established as pH 7.4 (buffer: PBS and 20 mM Tris-HCl (contains 20 mM NaCl before addition), respectively) with a distinct incubation time of 5 min and 10 min, respectively.
Figure 8A demonstrates the Hg
2+-induced aggregation and I
−-enabled anti-aggregation of N-1-(2-mercaptoethyl) thymine-capped Au NPs. TEM, DLS, and SPR analysis attested to both aggregation (in the presence of Hg
2+) and anti-aggregation by I
−. Linear ranges of I
− by mercapto-functionalized thymine and T-rich ssDNA on Au NPs were determined as 10–600 nM (at A
524/A
645) and 40 nM–4 µM (at A
520/A
650), with LODs of 10 nM and 13 nM, respectively. Both reports show high selectivity and exceptional recoveries (>89%) in real water and human urine samples; thus, they can be regarded as good works.
Figure 8. (
A) Schematic illustration of the analytical process for detecting iodide based on the anti-aggregation of N-1-(2-mercaptoethyl) thymine-capped Au NPs (reproduced with the permission from
[65]) and (
B) schematic illustration of the sensing mechanism based on the arginine-capped Au NPs and Cu
2+ (reproduced with the permission from
[68]).
Subsequently, the 2heDTC-Au NPs also aggregated and induced a color change from red to blue in the presence of Hg
2+. The aggregation was interrupted in the presence of I
− with a reverse color change from blue to red via anti-aggregation
[67]. The citrate on Au NPs were firstly modified via ligand exchange with 2-hydroxyethyldithiocarbamate (2heDTC), and then the interaction of Hg
2+ with 2heDTC resulted in aggregated bare Au NPs. When I
− was added, the interaction of 2heDTC to Hg
2+ was inhibited, and anti-aggregation occurred as supported by TEM, DLS, and SPR studies. The optimum pH and incubation time for this sensory study were fixed as pH 5 (buffer: 50 mM PBS) and 7 min. The linear regression of I
− was between 0.1 and 4 µM (at A
520/A
650), with an LOD of 20 nM. This work showed recoveries of >85% in edible salts; thus, it can be regarded as a unique colorimetric sensor for I
− quantification.
In this track, the arginine-capped Au NPs (Arg-Au NPs; particle size = 13 nm) were utilized for detecting I
− via anti-aggregation by engaging Cu
2+ (70 µM) as the aggregation-inducing agent
[68]. In the presence of Cu
2+, the Arg-Au NPs began to aggregate with a colorimetric response from red to blue via Arg-Cu
2+-Arg complexation. The dimeric Arg-Cu
2+ complex was disturbed by adding I
− to induce the anti-aggregation and produce a colorimetric response from blue to red. The actual reaction between Cu
2+ and I
− is represented by Equation (1) and schematically illustrated in
Figure 8B:
The best pH condition and incubation time were established as pH 7.4 (buffer: PBS) and 5 min. TEM, DLS, and SPR analysis also confirmed involvement of the anti-aggregation. The linear range of I
− was between 0.01 and 4 µM (at A
524/A
654), with an LOD of 10 nM. Drinking water and table salt investigations showed recoveries of between 96 and 108% and 94 and 103%, respectively, with RSD values of <3.9%. Though this work was effective in I
− quantitation, further optimizations on the Cu
2+ concentrations are still required in future work. By means of anti-aggregation, CI-Au NPs were engaged for the colorimetric detection of I
2 using thiosulfate (S
2O
32−; 5.94 mM) as an aggregation-inducing agent
[69]. Initially, Au NPs react with S
2O
32− to form a strong Au-S bond and to induce aggregation of NPs (with a color change from red to blue). Then a redox reaction takes place toward the formation of Au(S
2O
3)
23−, as described below in Equation (2):
Upon pre-incubating I
2 with Au NPs, the free thiosulfate is produced due to another redox reaction between I
2 and S
2O
32−, as described in Equation (3). Thereafter, anti-aggregation takes place to induce the color from blue to red.
TEM and SPR interrogations well attested the anti-aggregation strategy. The best result was achieved at pH 7 after 16 min incubation. The linear range of I2 was between 3 and 80 nM (at A519/A640), with an LOD of 1.36 nM. Moreover, plasma and tap water investigations showed >97% recovery. However, this work still needs more experimental data on the particle size variations, DLS, and interference studies.
Deng et al. described the anti-aggregation-enabled detection of thiocyanate anion (SCN
−) by engaging CI-Au NPs (particle size = 13 nm; conc. = 3.2 nM) and 8 mM of sulfuric acid (H
2SO
4) as the aggregation-inducing agent
[70]. In the presence of H
2SO
4 (8 mM), the Au NPs aggregated with a simultaneous color variation from red to blue. When SCN
− was added, anti-aggregation (via forming Au-S bonds) was induced to produce a blue-to-red color change, which was validated by SPR and TEM studies. The SCN
− displayed a linear regression of between 0.25 and 2 µM (at A
700/A
520), with an LOD of 0.14 µM. Intensity of the color was visible to naked eyes at 1 µM concentration. The tap water recoveries ranged between 94.8 and 101.3%, with RSDs of 1.6–3.2%. It should be noted that this work validated the selectivity of SCN
− over a wide range of cations and anions. However, further study is mandatory to improve the LOD toward nM or PM. Moreover, its applicability requires justification due to the use of an acidic aggregation-inducing agent. Song and co-workers described the anti-aggregation-enabled detection of SCN
− by employing CI-Au NPs (particle size = 13 nm) and cetyltrimethyl ammonium bromide (CTAB; 0.5 µM) as the aggregation-inducing agent
[71]. Due to the strong electrostatic interaction between CTAB and stabilizing agent citrate, the aggregation induced a color change from red to blue. When SCN
− was added, it strongly coordinated with the Au surface with a high stability constant; thus, Au NPs were re-dispersed via anti-aggregation and displayed a color change from blue to red. The SPR, color change, and nanoparticles size variations during subsequent interaction of CTAB and SCN
− are displayed in
Figure 9A,B.
Figure 9. (
A) UV−vis spectra and colors of Au NPs in the absence (a) and presence of 0.5 µM CTAB (b), after the addition of 0.5 µM CTAB and 1.5 µM SCN
− (c). (
B) TEM images of Au NPs, naked Au NPs (
a), and Au NPs with 0.5 µM CTAB: in the presence of 0 (
b), 1.5 µM (
c), and 2.0 µM SCN
− (
d); scale bars for (
a–
d) = 200 nm ((
A,
B) are reproduced with the permission from
[71]).
The probe had a high selectivity over a wide range of analytes and showed the best sensory performance to SCN
− at pH 7 (buffer: 10 mM PBS) after 15 min incubation. The linearity of SCN
− ranged between 0.1 and 1.5 µM (at A
650/A
520), with an LOD of 6.5 nM. Real studies on solid and liquid milk samples demonstrated a recovery of >90% with an RSD of ≤5.5%. Based on the selectivity, sensitivity, and real samples investigations, this work can be regarded as nice invention for SCN
− monitoring in real-time. By employing the 2-amino pyridine (2-AP; 50 µM) as the aggregation-inducing agent, CI-Au NPs (particle size = 10–44 nm; conc. = 8.3 nM) were engaged in detecting SCN
− [72]. In this work, the +vely charged 2-AP interacted with -vely charged CI-Au NPs to form the static electricity and to induce the aggregation with a color change from red to blue. Due to the strong binding affinity of SCN
− to Au NPs (via Au-S bond), the 2-AP-induced aggregation was disturbed, which resulted in the dispersion of Au NPs via anti-aggregation as evident from a color change from blue to red and SPR, DLS, and TEM studies. The optimum anti-aggregation induced by SCN
− occurred at pH 7 (buffer: 40 mM Tris-HCl) and displayed a linearity of between 0.4 and 1.2 μM (at A
675/A
520), with an LOD of 0.37 µM. Tap water investigations demonstrated recoveries of between 98.52 and 105.48%, with an RSD of <4.6%. Though the sensitivity of this work is high, further optimization is required to tune the LOD toward the nM level.
Lu et al. described the use of dithiothreitol (DTT; 4 µM) as an aggregation-inducing agent with CI-Au NPs (particle size = 13 nm; 11 nM) to quantify the hypochlorite (OCl
−) anion via the anti-aggregation strategy
[73], wherein the DTT with a free thiol unit at both ends enabled aggregation of Au NPs and displayed a color change from red to blue. Upon adding OCl
−, a redox reaction takes place to oxidize the DTT (6-electron transfer process) to its sulfonate derivatives, which results in anti-aggregation of Au NPs with a color change from blue to red, as depicted in
Figure 10A. The optimum condition for colorimetric detection of OCl
− was at pH 7.17 (buffer: 45 mM PBS), with incubation time of 2 min. Photographs and SPR, TEM, and XPS (XPS = X-ray photoelectron spectroscopy) studies attested to the anti-aggregation being induced by OCl
−. With the OCl
− concentrations between 0 and 7 µM, the SPR peak changes at A
665/A
520, with an LOD of 2 µM, could be visualized with naked eyes.
Figure 10B,C display the SPR and colorimetric changes as a function of the OCl
− concentration (0–8 µM). It should be noted that none of the interfering species could affect the OCl
− selectivity. Moreover, real water (tap water/lake water) investigations displayed a recovery >80%. However, this work lacks UV-based LOD calculations and DLS interrogations.
Figure 10. (
A) Schematic representation of the hypochlorite sensor with unmodified gold nanoparticles; (
B) optical absorption spectra in the presence of different concentrations of hypochlorite. Conditions: PBS (10 mM, pH 7.17), DTT (4.0 µM), and (
C) photographs showing the colorimetric responses (the hypochlorite concentrations, in µM, are listed on the centrifuge tubes) ((
A–
C) are reproduced with the permission from
[73]).
Liu and co-workers synthesized the mercapto–acetic acid–capped Au NPs (MA-Au NPs; particle size = 13 nm; conc. = 3 nM) and were employed in the detection of phosphate (PO
43−; Pi) anions via the anti-aggregation tactic
[74] in which the Eu
3+ (20 µM) was used as the aggregation-inducing agent. Adding the Eu
3+ to MA-Au NPs induced aggregation and a color change (from red to blue) because of the effective coordination with free carboxyl (-COOH) units of mercapto–acetic acid. Pre-mixing of Pi anion into the above mixed system inhibits formation of -COOH-Eu
3+ complex via strong Pi-Eu
3+ binding, as seen in
Figure 11. TEM and SPR studies confirmed the anti-aggregation, and the best pH and incubation time were determined as pH 7.4 (buffer: 25 mM Tris-HCl) and 5 min. The linear range of Pi was between 0.5 and 30 µM (at A
520/A
670), with an LOD of 76 nM. Real samples studies demonstrated selectivity over other interferences but lacked spiked recovery investigations. However, this is the only report available which is based on anti-aggregation of Au NPs.
Figure 11. Schematic representation of the colorimetric assay of Pi based on anti-aggregation of MA-Au NPs (reproduced with the permission from
[74]).
The CI-Au NPs (particle size = 13 nm) were also engaged in anti-aggregation-enabled detection of the nitrite anion (NO2−) using 4-Amino-thiophenol (4-ATP; 25 µM) as the aggregation-inducing agent. Herein, the 4-ATP induced aggregation (with a color change from red to blue) via a coating on Au NPs’ surface; however, the diazonium reaction with NO2− disturbed the aggregation and led to a blue-to-red color change. To attain the best result, the pH, H2SO4 concentration, and incubation time were fixed as pH 9.5 (buffer: Na2CO3–NaHCO3), 100 mM H2SO4 (catalytic amount of 10 µL from 1M stock) and 15 min, respectively. SPR, TEM, and interference studies validated the proposed tactic for NO2− quantitation. The linear range of NO2− was between 1 and 25 µM (at A685/A520), with an LOD of 1 µM. Spiked ground water and tap water investigations on the NO2− quantification showed recoveries of >98%, with an RSD of ≤8%, thereby confirming the practicality of this method. Based on the available data, this report can be regarded as an exceptional work for the NO2− assay.
4. Ag NPs-Based Colorimetric Assay of Diverse Analytes via Anti-Aggregation
Silver nanoparticles (Ag NPs) were also reported toward distinct analyte quantification via the anti-aggregation strategy similar to Au NPs, as discussed in this section. Duan et al. described the use of citrate-capped Ag NPs (CI-Ag NPs) toward the detection of Hg2+ via anti-aggregation in the presence of the aggregation-inducing agent 6-thioguanine (0.83 µM) [75], in which 6-thioguanine induced aggregation of Ag NPs to produce a color change from yellow to brown. However, the brown color was reversed to yellow via anti-aggregation by pre-mixing Hg2+ (at different concentrations). The underlying mechanism was that 6-thioguanine induced aggregation due to its strong electrostatic interaction toward CI-Ag NPs. However, the aggregation was inhibited by the presence of Hg2+ (via strong binding of 6-thioguanine with Hg2+) and resulted in the anti-aggregation of CI-Ag NPs. The best colorimetric response was achieved at pH 4 (100 mM HAc-NaAc) after 30 min incubation. The linear range of Hg2+ was between 0 and 333 nM (at A530/A394), with an LOD of 4 nM. SPR, TEM, interference, and spring water studies (recoveries of between 103 and 105%) validated this work as a major innovation. Figure 12A–C display the colorimetric response, SPR variations, and linearity of Hg2+ induced anti-aggregation of 6-thioguanine-CI-Ag NPs system, respectively.
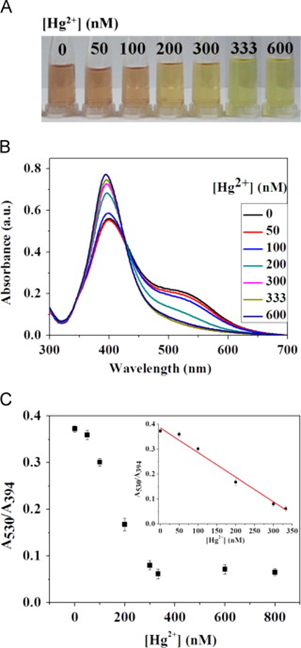
Figure 12. (A) The photographic images of the 6-thioguanine–Ag NPs solutions in the presence of different concentrations of Hg2+ (0–600 nM). (B) The corresponding UV–vis spectra of 6-thioguanine–Ag NPs. (C) The absorbance ratio (A530/A394) of 6-thioguanine–Ag NPs versus Hg2+ concentration. Inset to C: plot of A530/A394 versus the Hg2+ concentration. The concentration of 6-thioguanine was 0.83 μM ((A–C) are reproduced with the permission from [75]).
Anti-aggregation-enabled colorimetric assay of Cu2+ was demonstrated by employing CI-Ag NPs and engaging 1,4-dithiothreitol (DTT; 10 µM) as the aggregation-inducing agent [76], wherein DTT induced the aggregation of Ag NPs via a strong Ag-S bonding and displayed a color change from yellow to deep green. Upon pre-mixing Cu2+ in 2,6-pyridinedicarboxylic acid (PDCA, 5 mM), the DTT oxidized to form an intramolecular-S-S-bond, which inhibited aggregation and produced a reversed color change from deep green to yellow. The best result was attained at pH 2–10 (buffer: HCl-NaOH) after 20 min incubation. The linearity of Cu2+ was between 0.1 and 2 µM (at A408), with an LOD of 0.1 µM. SPR, TEM, interference, and real samples (tap water and ground water; recoveries of ≥95%, with an RSD of <8%) interrogations validated this method. Hence, it can be regarded as a remarkable research, but details on particle size variations are still missing.
He and co-workers reported the anti-aggregation-enabled colorimetric detection of Mn2+ by employing CI-Ag NPs (conc. = 0.28 nM) and L-Arginine (L-Arg; 0.7 mM) as the aggregation-inducing agent [77]. The Ag-N covalent interaction between Ag NPs and L-Arg caused aggregation, with the color of the solution changing from yellow to colorless. The color of Ag NPs changed back from colorless to yellow via anti-aggregation when the above solution was pre-treated with different concentrations of Mn2+. In fact, the anti-aggregation was attributed to the stronger attraction of L-Arg toward Mn2+ than that of the Ag NPs. The best result was obtained at pH 9.4 (buffer; BR) after 40 min incubation. Two linear ranges for Mn2+ were established between 0 and 700 nM and 5 and 70 µM (both at A390), with an LOD of 20 nM. As illustrated in Figure 13A,B, none of the competing species shows interference to Mn2+ selectivity. By this method, real water (lake, tap, and river) recoveries were >90%, with an RSD < 3%. This work is regarded as an exceptional innovation, but it still lacks particle size variations details.
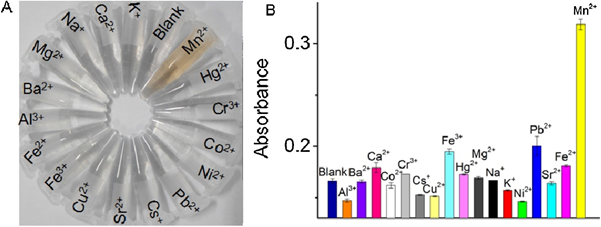
Figure 13. (A) The photographs and (B) the corresponding absorbance of the Ag NPs-l-arginine dispersions in the presence of different metal ions. The concentrations of both Mn2+ ions and other metal ions were 10 µM ((A,B) are reproduced with the permission from [77]).
Basiri et al. developed the Ag NPs–decorated graphene nanocomposites (rGO@Ag NPs; particle size = 3.7 ± 0.8 nm) for anti-aggregation-enabled colorimetric detection of Cu2+ using dopamine (DA; 25 µM) as the aggregation-inducing agent. The Ag NPs and 5 mg/mL of rGO was boiled at pH 10 and at 100 °C for 10 min to afford rGO@Ag NPs. The rGO@Ag NPs were well characterized by SPR, TEM, FTIR, XPS, and XRD studies (XRD = X-ray diffraction). Due to the H-bonding and π–π interactions between DA and rGO@Ag NPs, aggregation occurred (witnessed by TEM) with a color change from yellow to brown. In the presence of Cu2+, the interaction of DA to rGO@Ag NPs was hindered due to selective chelation of Cu2+ with DA and resulted in anti-aggregation (seen by TEM) with a color change from brown to yellow. Note that none of the competing species induces anti-aggregation, as shown in Figure 14. The best colorimetric response was observed at pH 7 (buffer: 10 mM PBS) after 15 min incubation. The linearity of Cu2+ ranged between 0.02 and 1.5 µM (at A405/A515), with an LOD of 9.8 nM. Moreover, real samples (human urine for DA and tomato for Cu2+) investigations demonstrated recoveries of >98%, with an RSD of <3%; thus, it can be noted as an excellent method.
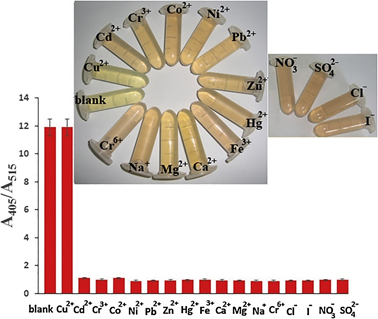
Figure 14. Colorimetric signals in the presence of different metal ions and anions (1.5 µM) as the interferences of Cu2+. Inset: the photographic images of the corresponding solutions (reproduced with the permission from [78]).
An anti-aggregation-enabled colorimetric assay of Br− and I− was demonstrated using CI-Ag NPs (particle size = 10–15 nm; conc. = 69.4 µM) and Cr3+ (125 µM) as the aggregation-inducing agent [79]. In the presence of Cr3+, the Ag NPs aggregated to produce a color change from yellow to brown. Due to the complexation of Cr3+ with Br− and I−, anti-aggregation occurred to reverse the color from brown to yellow. The linearity of Br– and I– was between 0.99 and 5.66 µM (at A500) and 0.99 and 4.16 µM (at A500), with LODs of 1.67 µM and 1.32 µM, respectively. Though this report was validated by SPR, DLS, and interference studies, data in TEM, pH, incubation time, and real-time investigations were insufficient; therefore, it can be only regarded as a preliminary work. To this track, the sulfanilic acid (SA) and catechol (CAT)–functionalized Ag NPs aggregate (SA-CAT-Ag NPs) was utilized for anti-aggregation-enabled colorimetric detection of F– [80]. The CA was firstly oxidized by silver ions followed by SA nucleophilic attack to deliver aggregated SA-CAT-Ag NPs with red color. By adding F– to the above mixture, it adsorbed on the Ag surface and stripped the SA-CAT stabilizer from -SO3− moiety, hence the aggregation was disturbed with a color change from red to yellow. The best color response was attained at pH 4–9 after 1 min incubation. The linear range of F– was between 1 and 40 µM (at A397/A508), with an LOD of 0.2 µM. This work was authenticated by SPR, TEM, interference (negligible), and spring water investigations. Thus, it can be noted as a nice approach for F– quantification.
Dong et al. demonstrated using poly-vinyl pyridine (PVP)–modified Ag NPs (PVP-Ag NPs; particle size = 6.24 nm) the colorimetric assay pyrophosphate ((P2O74−; PPi) via the anti-aggregation strategy [81]. Herein, the Pb2+ (5 µM) was employed as the aggregation-inducing agent to induce the PVP-Ag NPs (due to its crosslink effect with PVP) aggregation and to produce a color change from yellow to blue. Anti-aggregation was induced by pre-mixing PPi, which resulted in a colorimetric response from blue to yellow due to the chelating interaction between PPi and Pb2+. The best result was obtained at pH 5–9 at 20 °C after 30 min incubation. The linear ranges of PPi were between 0.2 and 2.0 μM and 2.0 and 10.0 μM (at A396), with an LOD of 0.2 µM. This work was authorized by smartphone device, SPR, TEM, statistical, theoretical, interference, real samples (tap water (recoveries between 79.6 and 107.6%) and canned meat (66.7–118.4%)) interrogations. Therefore, it can be regarded as an exceptional invention in Ag NPs-based colorimetric assay. Following a similar mechanistic approach, the 2-Mercapto-ethane sulfonate–modified silver nanoplates (MS-Ag NPls) were employed in the colorimetric detection of PO43− (Pi) on PADs in the presence of the aggregation-inducing agent Eu3+ (65.81 µM) [82]. In the presence of Eu3+, the color of MS-Ag NPls changed from pink to purple, which was reversed (from purple to pink) during the quantification of Pi. All the sensory experiments were conducted at pH 7 (buffer: 25 mM Tris) at an optimum incubation time of 3 min. The linear regression of Pi was between 10.42 and 313 µM (at A520), with an LOD of 3.44 µM. This work was also authenticated by water and soil investigations. Moreover, this is the only report available on the Ag NPls-based colorimetric assay. Therefore, it is regarded as a remarkable method.
The surface-enhanced Raman scattering (SERS) technique was employed with 4-mercaptopyridine (4-MPY)-modified Ag NPs (4-MPY-Ag NPs) for anti-aggregation-enabled detection of trypsin (an enzyme that aids with digestion) in the presence of the aggregation-inducing agent protamine (0.3 µg/mL (10 µL)) [83]. The presence of +vely charged protamine over 4-MPY-Ag NPs induced aggregation via strong electrostatic interaction with a color change from yellow to deep green. However, trypsin enhanced the enzymatic fragmentation of protamine, which resulted in anti-aggregation with a reversed color change from deep green to yellow. The SERS linear range was between 0.14 nM and 13.8 μM (at 1096 cm−1), with an LOD of 0.14 nM. All the SERS experiments were conducted at pH 7.4 (buffer: HEPES) within 1 min. Based on SERS, TEM, and interference studies, this work can be regarded as a unique one. An anti-aggregation-enabled colorimetric assay of poly diallyl-dimemethyl-ammonium chloride (PDADMAC; a coagulant in water and wastewater treatment) was proposed by employing CI-Ag NPs (particle size = 6 ± 2 nm) and 0.2 M PBS buffer (pH 7.4) as the aggregation-inducing agent [84]. The phosphate buffer induced aggregation of Ag NPs, which resulted in a color change from yellow to colorless. However, in the presence of PDADMAC, the Ag NPs were stabilized by electrostatic attraction and steric hindrance, which resulted in being dispersed NPs with a reversed color change (from colorless to yellow). Note that the colorimetric responses occurred within 3 min. The linear range of PDADMAC was between 1 and 100 mg. L−1 (at A396), with an LOD of 0.7 mg. L−1. This tactic was well authorized by SPR, TEM, interference, and tap water interrogations. Thus, it can be noted as a nice innovation. However, further optimization is mandatory toward the LOD reduction.
Though anti-aggregation-enabled Ag NPs-based colorimetric assays seem to be effective in analytes quantification, available reports are much less sufficient than that of aggregated Ag NPs-based colorimetric sensors [85][86][87][88][89][90]. Therefore, further research is required as suggested in perspectives.
5. Design/Sensory Requirements, Advantages, and Limitations
5.1. Probe Design and Sensory Requirements
The development of exceptional Au NPs- and Ag NPs-based colorimetric probes and their anti-aggregation-enabled sensory performances must follow the requirements as stated below:
- The selected label-free/modified Au NPs- or Ag NPs-based probes must possess dispersion stability in color and firm electrostatic charges to be able to interact with aggregation-inducing agents and to afford a certain degree of aggregation and a distinct colorimetric response.
- Selection of a suitable aggregation-inducing agent is essential to attain the primary colorimetric changes. An aggregation-inducing agent should have any of the following three characteristics: (i) analyte replaceable electrostatic interactions, (ii) analyte disposable interactive binding (such as Au-S, Ag-S, Au-N, Ag-N, etc.) over the NPs’ surface, and (iii) analyte coordinative units (such as thymine, -SH, -NH2, -COOH, -OCH3, and -OC2H5, etc.) that are able to form coordination complexes or undergo catalytic reactions to be released from the NPs’ surface. Moreover, it is essential to fix the concentration of the aggregation-inducing agent before taking further sensory interrogations.
- Optimizations of the effective pH conditions, suitable pH buffer solution, concentration, and maintenance circumstances are necessary for anti-aggregation-based colorimetric analyte detection.
- It is essential to fix the exact incubation time to achieve stable colorimetric response and to help to clarify the underlying mechanism and kinetics of the anti-aggregation.
- Though most of the anti-aggregation-enabled colorimetric sensory are conducted at room temperature, it is also important to fix the operative temperature toward real-time applications.
- To avoid complications in real water investigations, it is necessary to identify the interfering effect, allowed concentration, required masking agent, etc.
5.2. Advantages
Anti-aggregation-enabled Au NPs- and Ag NPs-based colorimetric assays have following advantages, as stated below:
- In general, anti-aggregation-enabled Au NPs- and Ag NPs-based analyte quantifications are conducted in an aqueous environment; therefore, they are tactics comparable to the available luminescent inorganic and organic nanomaterials-based sensors performed in diverse solvent conditions [122–130].
- The anti-aggregation-enabled Au NPs- and Ag NPs-based analyte detection can be observed with naked eye, thereby becoming a competing strategy to the reported organic dyes-based sensors [91][92][93][94][95][96][97].
- In the anti-aggregation-enabled sensory studies, sequential colorimetric detection of the aggregation-inducing agent and analyte can be carried out with reliable linearity and LODs down to nano- and pico-molar levels. Thus, it can be regarded as an advantage over other methods.
- By using the anti-aggregation-enabled Au NPs- and Ag NPs-based colorimetric assay, diverse analytes can be detected with specificity under a fixed pH value, incubation time, and operative temperature.
- Anti-aggregation of Au NPs in the presence of certain peptide sequences can be employed to avoid amyloid aggregation and Alzheimer’s disease occurrence.
- The anti-aggregation strategy in Au NPs- and Ag NPs-based colorimetric sensors can be effectively applied in real samples, such as water, soil, plasma, urine, etc.; thus, it can be noted as a great advantage toward development of the “state of the art” method.
5.3. Limitations
Anti-aggregation-enabled Au NPs- and Ag NPs-based colorimetric sensors also have a few limitations, as discussed below:
- Anti-aggregation-enabled Au NPs- and Ag NPs-based colorimetric sensory responses are aquatic environment-dependent; therefore, the detection of analytes in other solvent systems is not possible with this method.
- There are many optimization conditions (such as the aggregation-inducing agent, pH (buffer), NaCl effect, incubation time, temperature, etc.) required in order to attain the sensory responses, thereby limiting the frequent use of the anti-aggregation strategy.
- Due to the co-existence of the aggregation-inducing agent and analyte in the same mixture, the re-use of the mixture is not possible at all. Similarly, many reports used complicated assay protocols, which may have led to unknown human errors and restricted the use of this method.
- Elucidation of precise sensory mechanisms requires supportive evidence, such as FTIR, TEM, XPS, XRD, etc. Thus, this method was limited by available instruments and cost-effectiveness.
- The best result of anti-aggregation-enabled Au NPs- and Ag NPs-based colorimetric sensors can only be attained at a fixed interfering specie concentration, which is not possible with real samples (may contain large amounts of interference); therefore, real-time use of the strategy is still questionable.
- Real water/soil samples from different places contain various complicated matrixes. Thus, applying the anti-aggregation-enabled Au NPs- and Ag NPs-based colorimetric assay to all the real samples is not possible or may not deliver expected results.
6. Conclusions and Perspectives
In this entry, discussions on anti-aggregation-enabled Au NPs- and Ag NPs-based colorimetric sensing of metal ions, anions, bio-analytes, pesticides, and herbicides are delivered in great details. The assay protocols, optimized concentrations of aggregation-inducing agents, pH (buffer solution), incubation times, and operating temperatures are provided and tabulated for readers. The exact underlying mechanisms of each probe leading to the colorimetric response are explained with clear evidence. Real-time applications are illustrated, and comments are given on the performance of each individual report. Finally, researchers suggest the probe design/sensory requirements together with the merits and limitations of the anti-aggregation strategy. Though the anti-aggregation strategy-based colorimetric sensors are noted as being great innovations, there are still a few perspective points that need to be focused on, as noted below:
- Most reports on anti-aggregation-enabled Au NPs- and Ag NPs-based colorimetric sensors used complicated assay protocols, which should be simplified toward “state of the art”.
- Performance of anti-aggregation-enabled Au NPs- and Ag NPs-based colorimetric sensors depends on the pH values and buffer used. The use of unified pH buffer solution must be justified in the future to achieve a unique anti-aggregation strategy.
- By means of anti-aggregation, numerous reports are available on Au NPs-based colorimetric assay of Hg2+; however, none of them were commercialized toward practicality. This requires more efforts in future.
- The majority of anti-aggregation-based colorimetric sensors by Au NPs and Ag NPs focused on single-analyte quantification so far, which must be upgraded to multi-analyte detection. For example, the aggregation-inducing agent Hg2+ also has the coordination tendency for biothiols and I−, hence it can be employed in the quantification of both analytes.
- In many reports, anti-aggregation due to coordination of aggregation agents with analytes was not supported by valid evidence, which requires further clarification.
- Currently, anti-aggregation-enabled Au NPs- and Ag NPs-based colorimetric sensors are only available for the detection of limited metal analytes (such as Hg2+, Cu2+, Ag+, Mn2+, and Sc3+), thereby requiring more attention toward developing discrimination of more metal ion species.
- Anti-aggregation proposed for oxidative catalytic reactions falls short of evidence, and it should be addressed with more experimental data.
- Concerning the social impact, anti-aggregation-enabled in vitro colorimetric assay of amyloid β peptide toward the inhibition of Alzheimer disease requires great attention in future research work.
- Reports on anti-aggregation-enabled Au-NPs colorimetric assay of anions, pesticides, and herbicides are insufficient to justify the effectiveness of the strategy toward those analytes. This issue should be addressed in future.
- Only a few reports are available on the anti-aggregation-stimulated Ag NPs-based colorimetric sensors toward diverse analytes detection. Therefore, research on anti-aggregation of Ag NPs toward the discrimination of distinct analytes requires great attention.
- Compositions of Au NPs and Ag NPs with other established luminescent nanomaterials can be the focus to afford anti-aggregation-based colorimetric and luminescent responses toward specific analytes.
- Many anti-aggregation-based sensory reports lack theoretical supports, which should be addressed using density functional theory (DFT) investigations in future.
Though there are still many unclearly issues requiring further clarification, colorimetric recognition of specified analytes via anti-aggregation is becoming an important research field. Currently, there are many groups working on the development of new anti-aggregation-enabled Au NPs- and Ag NPs-based colorimetric sensory probes to remedy the aforementioned issues. However, the anti-aggregation strategy can be regarded as exceptional for analyte quantification in terms of naked eye detection ability and real-time applicability.