The process of the pulsed-laser-induced quenching of liquid-solid interface reactions and pulsed laser evaporation to form composite films were then covered in depth, which provided a theoretical framework for the creation of new techniques for the synthesis of nanostructures. The laser irradiation process in a liquid environment, also known as laser ablation in liquid (LAL), can greatly improve ablation efficiency. The function of the liquids, such as water, involves heat transfer (conduction or convection) and bubble movement, which aids in the removal of the ablation particles that have been redeposited on the surface of the material and inhibits the fragmentation of chip oxidation. In addition, the liquid environment effectively cools the targets, preventing excessive heat buildup. Therefore, LAL can be widely used in laser cleaning and laser shock peening.
1. Laser Ablation Mechanism
The laser ablation of solid targets in a liquid environment allows for the generation of NPs with a variety of valuable properties, such as high-purity, easily functionalized surfaces, metastable compositions, or complex structures, including doped nanocrystals, core-shell, hollow microspheres, nano frills, or nano fluorescents
[1]. At the same time, LAL has the following advantages: environmental sustainability, a simple experimental setup (no need for extreme conditions of a synthetic environment), and the lasting stability of nanoparticles, which are completely free from harmful pollutants or dangerous synthetic reactants.
[2][3]. Au and Ag NPs were chosen as case studies because they are very important tools in nanotechnology, especially because of their optical and catalytic properties, the multiple possibilities of surface (biological) conjugation, chemical stability, and biocompatibility
[4]. Furthermore, in recent years, there has been a lot of interest in the laser-assisted synthesis of Au and Ag NPs, and these nanoparticles can be used as reference materials for LAL.
Many physicochemical reactions occur during the formation of NPs during the LAL process, which can be roughly divided into three categories
[5]. Mechanism A mainly postirradiates the colloidal mixture of the previously generated Ag-Au-NPs; mechanism B is the direct formation of Ag-Au-NPs during ablation; mechanism C is adsorbed by the bulk material through the incident laser pulse to form a plasma (C2), subsequently generating expanded cavitation bubbles, while crystalline NPs (C1) are formed inside the cavitation bubbles through nucleation and coalescence.
The most important difference between laser-ablating solids in a vacuum or dilution gas and liquid ablating is that liquid restricts the movement of the plasma plume. Therefore, a series of processes, such as the generation, transformation, and condensation of the plasma plumes generated by solid laser ablation in a liquid environment, have taken place under the constraint of liquid. Importantly, the constraints of the liquid will greatly affect the thermodynamic and dynamic properties of the evolution of the plasma plume, resulting in a different environment for the formation of the condensed phases of the laser-ablated solids in a vacuum or diluted gas
[6].
When the laser ablation of a solid target produces nanoparticles in the liquid, the temperature rise of the liquid will have a certain influence on the formation of cavitation bubbles and particles. Menendez et al.
[7] analyzed the effect of temperature on the hydrodynamic diameter of nanoparticles. The effect of the physical properties of the liquid on the hydrodynamic diameter of the nanoparticles was investigated. Ageev et al.
[8] found that laser-etched cavities at long pulses (1 ms) or high pulse rates (50 kHz) were wide and produced shallow bubbles and absorbed debris due to light scattering. Dell’Aglio et al.
[9] discussed the mechanisms involved in LAL technology in detail based on some basic experiments. After laser-matter interaction, laser-induced breakdown occurs first, followed by the formation of a shock wave
[10], plasma expansion and cooling, and finally, cavitation bubble formation, expansion, and collapse
[11]. When the bubble breaks, the nanoparticles generated in the plasma-cooling phase will diffuse into the surrounding liquid, forming a colloidal solution. The relationship between the primary plasma and the bubble was examined by Tamura et al.
[12], and they discovered that the pulse duration had a significant impact on the amount of plasma quenching during the first stage. When the pulse duration was the longest, i.e., 100 ns, a narrow spectral line was obtained. It could be seen from the plasma emission image that the plasma emission intensity obtained by a 100 ns pulse was higher than that obtained by a 30 or 50 ns pulse. After 600 ns of pulse irradiation, the bubble size obtained by different pulse durations was basically the same. The emission was observed below the target surface in the image. This was the reflection of light on the target surface. It was difficult to observe the optical emission of the plasma in a direction that was completely parallel to the target surface.
2. Size and Shape Control
Research on laser-fabricated nanoparticles in liquid environments focuses on the characterization of nanoparticles, especially their size and shape. The size and shape of nanoparticles directly affect their properties (catalytic performance, surface activity, etc.). So far, many metal nanoparticles have formed reliable preparation methods, but understanding how to control the size and shape of nanoparticles will be a major focus and challenge in future research.
Size control: The existing research mainly focuses on the high reaction characteristics of nanoparticles to control their size. Henglein et al.
[13] created gold NPs in early research into laser-based nanoparticle synthesis via the ablation of gold thin films at a wavelength of L = 694 nm using a pulsed ruby laser (sized between 5 and 15 nm). Mafune et al.
[14] produced colloids of silver (10 nm particle size) and gold (8 nm particle size) using a Nd: YAG laser with a pulse duration of t = 10 ns, and a wave-length of L = 532 nm. By introducing chloride into the liquid environment during the laser ablation, Prochazka et al.
[15] were able to avoid the production of larger colloidal particles and reduce the average particle size (11 nm, silver). Polyvinylpyrrolidone (PVP) can increase the formation efficiency and stability of Ag NPs, as shown by Tsuji et al. in their study
[16]. Tomko et al.
[17] revealed that the height of the liquid column and the ambient pressure have a greater impact on the maximum bubble radius and collapse time, thereby affecting the particle size distribution of nanoparticles. The size of nanoparticles prepared by laser in a liquid environment is mostly below 100 nm, which can achieve size control below 100 nm.
Shape control: Utilizing lasers to process nanomaterials allows for better shape control. Setoura et al.
[18] used optical spectroscopy and scanning electron microscopy (SEM) to measure the morphological changes in single Au nanoparticles under the excitation of a focused 488 nm continuous laser. The heating rate under continuous laser irradiation is much lower than that under pulsed laser irradiation, which allows for better control over the heating and morphology changes of nanoparticles. In summary, laser nanoparticle preparation in a liquid environment can provide good size and shape control.
Table 1 summarizes the shape and size of Au/Ag NPs in the typical literature in recent years.
Table 1. Comparison of the size and shape of NPs in LAL.
NPs |
Methods |
Size (nm) |
Shape |
TEM Images |
References |
Ag |
LAL |
100–200 nm |
Spherical |
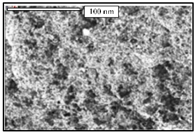 |
[19] |
Ag |
LAL |
5–140 nm |
Spherical |
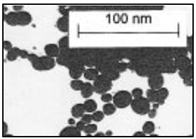 |
[15] |
Ag |
LAL. |
1.66 ± 0.37 nm |
Catenary |
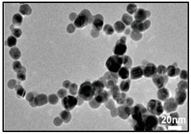 |
[20] |
Au |
LAL |
20–40 nm |
Spherical |
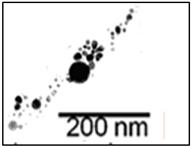 |
[7] |
Au |
LAL |
20 nm |
Spherical |
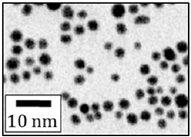 |
[16] |
Au |
LAL |
20–30 nm |
Spherical |
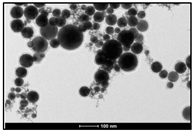 |
[21] |
Au |
LAL (nanosecond pulsed) |
20 nm |
Fractal structures |
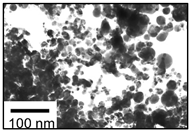 |
[3] |
3. Summary
This section mainly reviews the main preparation methods (LAL) of gold and silver nanoparticles, preparation principles and mechanisms, and the influence of shape and size control over nanoparticles. Size control of noble metal nanoparticles prepared by laser ablation can be achieved by adding specific molecules to the preparation environment in water that physically or chemically interact with the particle-forming surface to limit the growth of nanoparticles (Ag, Au). It was found that the particle size of noble metals (Au, Ag) was successfully limited by using ionic surfactants
[22], sodium chloride
[19], etc. However, the specific mechanism that limits particle growth requires further study. In conclusion, LAL-prepared nanoparticles (gold, silver, etc.) are well characterized but still lack size control and stability, especially in pure water. In addition, the physical and chemical properties of the surface of NPs are closely related to their application scenarios, which require in-depth research.