1. Chitosan
Chitin is the most abundant natural aminopolysaccharide polymer, being the structural component that confers resistance to exoskeletons of crustaceans, insects, and the fungal cell wall. CTS is obtained by enzymatic or chemical deacetylation of chitin. It is a linear polysaccharide consisting of
N-acetyl-2-amino-2-deoxy-D-glucose (
N-acetyl-D-glucosamine) and 2-amino-2-deoxy-D-glucose (D-glucosamine) units
[1]. Chitin and CTS are versatile biomaterials, similar in their properties to cellulose. Due to its solubility and the reactivity of free amino groups (-NH
2), CTS is a useful material in various fields, having remarkable characteristics such as biocompatibility, low toxicity, biodegradability, film formation capacity, etc.
[1][2][3][4].
Nanoparticles (NPs) of folate-CTS conjugated with DOX and pyropheophorbide acid has been prepared using a tripolyphosphate-assisted ionotropic gelation method. CTS-containing NPs are hydrophilic, possess good biocompatibility and can improve membrane permeability. At the same time, folic acid (FA) functions as a ligand for targeting the cell membrane
[5]. There is a specific receptor, namely the folate receptor (FR) which is a membrane protein with a high affinity for folate binding and transport. FR is overexpressed on the surface of neoplastic cells, in the ovaries, kidneys, colon, uterus, and lungs, thus becoming a potential target for tumor therapy
[6][7][8]. The FR family is composed of three glycosylphosphatidylinositol-anchored membrane glycoproteins (FRα, FRβ and FRγ–identified in human tissues), rich in cysteine, capable of transporting drugs in the cytosol by endocytosis
[9][10]. FR isoforms are considered promising therapeutic targets due to frequent overexpression of FRα in cancer cells of epithelial origin and FRβ in macrophages of tumors
[11][12]. FRα has a high affinity for folates that are not usually included in food, becoming available for conjugates of FA with anticancer drugs
[13]. The importance of the FR in the development and progression of cancer has determined the intensive study of FR-targeted therapeutic approaches
[14]. Because FR transports folate through a high affinity endocytic pathway, a variety of antifolate drugs targeting FR and folate conjugates that use this transport mechanism, including cytotoxic drugs, have been developed
[15][16][17]. The mechanism of endocytosis of folate–drug conjugates mediated by FR. The reduced folate or FA binds to FR and the receptor–conjugate complex is internalized by endocytosis, followed by the release of the drug
[18][19][20][21]. To make the most of this effects for antitumor therapy, a series of compounds were developed among which: FA conjugated to macromolecules
[22], NPs
[23][24][25], and liposomes
[26].
CTS in the form of water-soluble and biocompatible NPs, was conjugated with cytotoxic methotrexate molecules (MTX) by means of hydroxyl and amino functional groups
[27]. CTS NPs have been attached to terbium and rare metal atoms as carriers for MTX. CTS functionalized luminescent rare earth doped terbium NPs as a drug-delivery system for MTX were studied. There has been a significant increase in the efficiency of CTS NPs loaded with MTX compared to the free drug
[28].
Numerous biomaterials such as CTS-based nanoparticles have been recently developed for reasons related to the remarkable attributes of this natural polymer, as one of the most promising delivery vehicles for chemotherapy and cancer diagnosis, due to its unique characteristics: biodegradability, biocompatibility, enhanced penetrability of the cell membrane, high transport capacity of drug molecules, ability to have a multifunctional, and a prolonged circulation time
[29].
The use of this natural polymer as a nanomaterial in the administration of drugs contributes to the improvement of the pharmacokinetic profile of the active drug molecule by increasing the cellular absorption of poorly soluble drugs
[30][31]. It improves the bioavailability of the drug at effective doses.
Several studies have shown that the overall shape and appearance of NPs and their size contribute decisively in the distribution of the active drug molecule
[32][33]. Balancing the size and geometry of NPs is essential for the efficiency of drug delivery, in order to achieve a prolonged systemic circulation time, thus improving their biodistribution and pharmacodynamics
[34]. In the in vitro cytotoxicity assay, the positive charge of NPs has an important role in the uptake of the conjugate and in consequence of the active drug molecule into the tumor cells, demonstrating increased cell absorption and drug release into cells
[35]. The absorption efficiency of these polymers is closely related to the properties of NPs (shape, particle size, hydrophobicity, and surface charges)
[36][37][38][39]. NPs of polysaccharides also have excellent advantages in the transport of antitumor drugs
[40][41][42].
Gemcitabine (GEM), a nucleoside analogue that is effective in a significant number of malignancies, has many limitations, such as reduced half-life leading to more frequent administration, low oral bioavailability, which limits the antineoplastic potential of this drug and increased toxicity
[43]. Studies have been performed to design a vector in order to reduce the burden of frequent dosing and high toxicity associated with the use of GEM. Thus, NPCTS NPs encapsulating GEM have been developed and studied in vitro, ex vivo and in vivo
[43][44]. Moreover, a peptide conjugate from CTS derivative, trimethyl CTS—and a cysteine-serine-lysine-serine-serine-aspartic acid-tyrosine-glutamine-cysteine peptide capable of improving the oral bioavailability of GEM as a consequence of its specificity to target intestinal cells and promote cell absorption have been developed
[43].
Theranostics is a therapeutic strategy that uses a combination of a radioactive substance for diagnosis and an active therapeutic ingredient against neoplasms or metastases. Thus, theranostic agents are multifunctional agents composed of a payload carrier, therapeutic agents, and a ligand for targeting the complex
[45][46]. The use of CTS as a theranostic agent in imaging investigations and it is based on its exceptional functional characteristics: positive charge at slightly acidic pH, biocompatibility, biodegradability, and low immunogenicity, which make it a multifunctional agent
[47][48][49][50][51].
Thus, a complex of gold and CTS nanoclusters has been molded into theranostic NPs for bioimaging and suicide gene therapy
[52]. Gold nanoclusters provided optical imaging properties. In addition, genes in the suicide-gene therapy strategy generate a bifunctional enzyme (cytosine deaminase-uracil phosphoribosyltransferase), which is involved in the transformation of the prodrug 5-fluorocytosine into the active cytotoxic molecule 5-fluorouracil (5-FU) in cervical neoplasm. In this case CTS stabilizes the theranostic NPs in optical emission. Structural investigations revealed the formation of the nanocomposite by binding gold atoms to the amino and hydroxyl groups of the CTS molecule, and the incorporation by encapsulation of the cytotoxic molecule of 5-FU was 96%
[53].
In addition, studies have been performed to increase the efficiency of the 5-FU molecule coupled with the hyaluronidase (Hase) enzyme system incorporated into spherical CTS-NPs (5-FU-CTS-NPs) using three-dimensional (3D) spheroid culture HCT-116 (colorectal carcinoma cell line). Hase-loaded NPs (CTS-NPs) have been used in recent studies to enhance cancer treatment efficacy. It has been found that the use of the Hase enzyme increases the drug infusion in the tumor tissue. These CTS-NPs have been shown to increase the ability to deliver and enhance the anticancer activity of 5-FU in HCT-116 3D culture cell studies
[54].
A CTS-based nanocomplex with lactobionic acid has been developed to target the sgVEGFR2/Cas9 plasmid and paclitaxel (PTX) as cytotoxic active molecule in the treatment of liver carcinoma. The study reported the tumor accumulation of the complex and the in vivo stability of the nanosystem. Furthermore, gene–drug loaded NPs promote the anti-tumorigenic pathway by suppressing pro-inflammatory cytokines (IL-6, IL-8) and protein expression in tumor angiogenesis (NF-κB p65), highlighting the potential of PTX when combined with gene therapy for overexpression of vascular endothelial growth factor 2 (VEGFR 2) on HCC cells, as a basis for synergistic gene–chemotherapy
[55][56].
To date, there is a reduced number of studies on the use of CTS derivatives and other polymers for the delivery of genome editing components
[57][58]. A CTS-negative fluorescent protein was encapsulated to deliver Cas9 protein and sgRNA. To obtain the Cas9 protein complex—CTS (
Figure 1), polyglutamic acid was used to modify the protein. A 177 nm NP was formed by polyplexes composed of CTS, red fluorescent protein, a derivative of Cas9 protein and sgRNA specific for glutathione peroxidase-4
[57].
Figure 1. Nanoassembly of fluorescent protein−CTS Cas9/sgRNA and gene−donor. Adapted from
[57], MDPI, 2020.
2. Hyaluronic Acid
Hyaluronic acid (HA) is a key compound of the extracellular environment, present primarily in the vitreous humor and in cartilage. It is a natural polysaccharide, first isolated in the 1930s from the vitreous humor of the bovine eye by Meyer and Palmer
[59][60]. Structurally, it is a unique natural polysaccharide, with a linear structure of repeated disaccharide units, composed of D-glucuronic acid and
N-acetyl-D-glucosamine
[60][61].
HA has functional groups that can be used for different conjugations or can undergo various modifications, which makes this natural polymer a major component of multifunctional NPs useful in various therapeutic strategies
[62][63][64][65][66].
As a consequence of its high compatibility, low toxicity, and biodegradability, HA is also extensively studied as a conjugating agent in PDEPT. In addition to these properties, HA can bond overexpressed receptors specific to tumor cells, so it can be used for targeting antineoplastic drugs. Therefore, HA has attracted a lot of attention as a vehicle for drug delivery
[67]. HA has been shown to be an important carrier of drugs targeted to tumor cells. It shows important advantages such as:
- -
-
the use of lipid NPs with adequate HA coating as carriers of biocompatible drugs is an effective means of delivering the drug and at the same time significantly reduce side effects;
- -
-
improved distribution;
- -
-
improved release of drugs in cancer cells due to its high potential of targeted chemotherapy for tumors with increased CD44 receptor expression;
- -
-
enhanced efficacy
[68][69][70].
At the molecular level, HA interacts with certain cell surface receptors, such as CD44, LYVE-1 (lymphatic vessel endothelium receptor-1), and RHAMM (receptor for hyaluronan-mediated motility), which are usually overexpressed in tumor tissue
[66][71]. Following the linkage of HA to the CD44 receptor the internalization of this complex by endocytosis. HA is released and degraded by enzymatic reaction to low-molecular-weight components
[65]. Thus, CD44 (an ubiquitous glycoprotein) has great potential to be an active target receptor as a consequence of overexpression in breast, colon, ovarian neoplasm, and squamous cell carcinoma
[72]. HA is used to obtain conjugates for targeted drug delivery at specific sites due to its strong affinity for CD44. A series of advantages are expected following the conjugation of HA to cytotoxic agents regarding aqueous solubility, distribution, and efficacy
[73].
Several developed HA conjugates are presented below:
Thus, a HA-AMINO ACID-PTX conjugate was studied. An amino acid acting as a crosslinker, or a linker was used in this conjugate: the carboxyl group of the amino acid is linked to the hydroxyl group of the cytotoxic drug molecule PTX and then the amino group of the amino acid was linked to the carboxyl group of HA to obtain a conjugate of hyaluronic acid-amino acid-PTX (
Figure 2)
[74]. In the aqueous solution, the self-assembled amphiphilic conjugate into NPs and the active PTX molecule was surrounded by a hydrophilic HA structure. The active drug PTX was released by esterase mediated hydrolysis
[75][76].
Figure 2. Drug–hyaluronic acid conjugates; (
a) HA-amino acid-PTX conjugate [
119]; (
b) HA-DTX [
125]; (
c) HA-5-FU adapted after [
129]. 5-FU—5-fluorouracil; AA—amino acid; DTX—docetaxel; HA—hyaluronic acid; PTX—paclitaxel.
Numerous types of nanostructures have been studied, such as lipid-, carbon-, and polymeric-based NPs, that have been modified with HA to improve the delivery of cytotoxic molecules, such as DOX, to cancer cells
[77]. A novel multifunctional system, that may be used for both tumor-targeting drug delivery and imaging, was developed through the assembly of a HA-DOX conjugate with a cationic conjugated polymer, represented by poly {[9,9-bis(6′-(
N,
N,
N-diethylmethylammonium)hexyl)-2,7-fluorenylene ethynylene]-
alt-
co-[2,5-bis(3′-(
N,
N,
N-diethylmethylammonium)-1′-oxapropyl)-1,4-phenylene]} tetraiodide (PFEP). In vitro release of the active molecule DOX, from the PFEP/HA-DOX complex, took place in the presence of hyaluronidase (Hase) within the first 15 min and reached a plateau after 25 min
[78][79].
Another macromolecular conjugate, HA-Docetaxel (DTX), was developed with the aim of improving the pharmacokinetics and pharmacodynamics of DTX (a semisynthetic analogue of PTX). Drug release, cytotoxicity, cell absorption, cell cycle inhibition, and subacute toxicity were studied. In this sense, tumor cell lines that overexpressed CD44 receptors, such as human breast cancer cell lines MCF-7 and MDA-MB-231 were used
[80][81]. 1-ethyl-3-(3-dimethylaminopropyl) carbodiimide/N-hydroxysuccinimide coupling chemistry was used for the activation of the COOH group of HA before conjugation. The obtained HA-DTX conjugate was characterized by spectral and thermal analysis
[82].
HA-Cisplatin (
cis-diamminedichloroplatinum (II), CDDP) is a conjugate that has been shown to reduce the significant side effects that limit the use of cisplatin. Through this conjugation there is an increase in the concentration of cisplatin in the lymphatic vessels, an increase in the distribution at the tumor site, a reduction in systemic toxicity and especially renal toxicity and there is an early inhibition of tumor metastases
[83]. In order to efficiently deliver the CDDP-active molecule, especially in neoplastic ovarian tissue, titanium dioxide NPs have been designed as a nanometer-sized solid vector. TiO
2 NPs were conjugated to HA, forming HA-TiO
2 NPs that specifically target the ovarian cancer cells. HA-TiO
2 NPs were loaded with CDDP to achieve a tumor-targeted drug-delivery system
[84].
HA-5-FU is a conjugate in which the carboxylic group of HA binds to 5-FU through adipic acid dihydrazide and succinic anhydride linkers. An increase in the antiproliferative activity of HA-5-FU conjugate was observed on various cancer cell lines
[85][86].
HA layer-by-layer NPs were developed in order to improve the efficacy of cytotoxic molecules and reduce their toxicity
[87]. A new CTS-based HA hybrid polymer conjugate containing irinotecan and 5-FU was obtained in which irinotecan and poly(D,L-lactide-
co-glycolide) represent the central core, CTS, and 5-FU the adjacent part and HA the outer layer. The in vitro and in vivo antiproliferative activity of the modified conjugate was superior to unmodified NPs, single-drug loaded NPs, or free drug, thus providing a promising strategy for targeted gastric cancer therapy
[88][89][90][91]. Systems such as lipid–polymer hybrid NPs (LPNPs) with modified HA consisting of polymeric cores and lipid shells combine the advantages of polymeric NPs with those of lipid NPs or liposomes, increasing their physical stability and biocompatibility. Thus, HA modified, irinotecan and gene co-loaded LPNPs (HA-I/D-LPNP) were obtained and evaluated in vitro and in vivo on colorectal cancer cells and cancer-bearing mice. The conjugate system showed the highest antitumor activity and the best in vivo transfection efficiency, proving the beneficial effects of the targeted combination therapy
[92].
3. Dextran
DEX is a natural polysaccharide studied as a polymeric carrier in targeted drug-delivery systems. From a structural point of view, DEX is a polysaccharide consisting of α-(1→6) and α-(1→3) glycosidic bonds of variable lengths (3–2000 kDa), which form a linear polymer through 1,6-glycosidic bonds with a certain degree of branching through 1,3-glycosidic bonds. The structure of DEX contains hydroxyl groups (OH) and terminal aldehyde groups (CHO), which can be chemically modified in order to obtain DEX-based biomaterials for various biomedical applications
[93][94]. The hydroxyl groups are very important for conjugation with other substrates.
DEX in the form of NP microspheres has been used to improve the solubility of antitumor drugs and at the same time to improve their distribution at the target site of action
[95][96]. DEX microspheres have many advantages, such as: biodegradability and biocompatibility, as well as non-immunogenicity and non-toxicity, all important factors for clinical applications. Regarding their physico-chemical profile, these microspheres are advantageous because they are easy to filter, are neutral, and soluble in water. Also, the functional groups of DEX provide promising advantages for biological and imaging applications
[97][98][99][100]. Thus, a controlled-release system based on DEX microspheres has been developed, the vehicle of choice for the administration of mitomycin C, a potent promoter agent, which works by bioreductive activation in antineoplastic therapy
[101].
Stimuli-sensitive covalent PDCs are promising alternatives to polymer NPs that rely on physical drug encapsulation, due to their advantage of providing much more precise control over the active drug dosage and release
[102][103][104]. The unique ability of PCDs to self-assemble into NPs and stimuli-sensitive drug release are among the advantages of this NP-based drug-delivery systems
[102][105][106][107].
The conjugate between DEX and PTX through a disulfide linker. DEX-S-S-PTX PDC demonstrated significant cytotoxicity on BT-549 and MCF-7 tumor cells, with the release of PTX in response to the intracellular reducing medium of tumor cells. DEX-S-S-PTX NPs showed IC50 (half-maximal inhibitory concentration) values and mechanism of action similar to those of free PTX, emphasizing the intracellular release of the drug
[108][109].
Due to its hydrophilic, biocompatible, and biodegradable properties, DEX has been used to obtain a conjugate with another cytotoxic drug, such as MTX. MTX is linked to DEX by a peptide that can be cleaved by matrix-metalloproteinase-2 (MMP-2) and matrix-metalloproteinase-9 (MMP-9), two important enzymes present in tumor tissue. Its antitumor efficacy and systemic side effects were evaluated in vivo and were compared with free MTX and a similar conjugate with a different linker, insensitive to MMP enzymes, in doses equivalent to intraperitoneal administration, that have been released in tumor tissue and not in a healthy tissue. This research showed that DEX-MTX conjugate has been effective in releasing the drug into tumors that express the specific enzymes MMP-2 and MMP-9, which are characteristic of malignant tumors. The linker used is a peptide structure consisting of the following amino acids: glycine–isoleucine–valine–glycine–proline–leucine
[110].
The in vitro and in vivo antitumor activity of DOX encapsulated NPs using deoxycholic acid (DA) conjugated dextran (DEX-DA) was evaluated on CT26 colon cancer cell line. The DEX-DA nanoparticles on which the DOX molecules have been incorporated had spherical shapes and particle sizes between 50–200 nm. In addition, the rate of drug release was accelerated in acidic pH compared to the alkaline pH. The results of the cytotoxicity assay using CT26 cell line, showed that the NPs possessed increased antitumor activity compared to free DOX
[111].
Another approach for targeting the active DOX molecule is in the form of NP in the DEX-FA polymer conjugate (DEX-FA-NPs). The FR-mediated endocytosis and in vivo targeted delivery have been demonstrated for DEX-FA-NP, with an increased antitumor activity and reduced side effects
[112].
Another approach is that of magnetic NPs combined with different materials for various magnetically guided drug-delivery systems that can be used for targeted administration of cytotoxic drugs. For example, transferrin conjugated magnetic DEX-spermine NP (DS-NP) was developed for blood brain barrier penetration of drugs
[113]. On the other hand, DEX-coated superparamagnetic iron oxide NPs (DSPIONs) have been developed as carriers for cytotoxic molecules due to their biocompatibility and ability to reduce the toxicity of some cytotoxic molecules, such as DOX
[114][115].
Other strategies for obtaining NP conjugate systems with different pH-sensitive cytotoxic molecules have been approached
[116][117][118]. An example is represented by the covalent conjugation of oxidized DEX (oDex) with two cytotoxic molecules, Pt (IV) prodrug and DOX, with the formation of oDex-Pt + DOX NPs with an average diameter of approximately 180 nm, characterized by a pH and reduction dual sensitivity. The efficient cellular absorption of oDex-Pt + DOX NPs in human cervical carcinoma HeLa cells was identified using confocal laser scanning microscopy. The antitumor activity of oDex-Pt + DOX NPs was similar to the combination of the free drugs, but the oDex-Pt + DOX NPs displayed an improved ability to reverse tumor resistance in cisplatin-resistant A549 lung cancer cells
[119][120].
4. Pullulan
PL is a natural polysaccharide, an exopolysaccharide, composed of maltotriose units, biocompatible biopolymer, biodegradable, non-toxic, nonmutagenic, and noncarcinogenic [121]. It is a product of aerobic metabolism of a species of fungus Aureobasidium pullulans [122]. Structurally, this linear natural polymer consists of three units of glucose, linked by an α-1,4 glycosidic bond, the units of maltotriose thus formed being linked by an α-1,6 glycosidic bond. PL is obtained naturally from starch under the action of the polymorphic fungal species Aureobasidium pullulans [123][124].
PL is a natural polysaccharide, an exopolysaccharide, composed of maltotriose units, biocompatible biopolymer, biodegradable, non-toxic, nonmutagenic, and noncarcinogenic [121]. It is a product of aerobic metabolism of a species of fungus Aureobasidium pullulans [122]. Structurally, this linear natural polymer consists of three units of glucose, linked by an α-1,4 glycosidic bond, the units of maltotriose thus formed being linked by an α-1,6 glycosidic bond. PL is obtained naturally from starch under the action of the polymorphic fungal species Aureobasidium pullulans [123][124].
5. Heparin
HEP is a natural polymer and could be considered an alternative for drug delivery in cancer cells. A HEP-FA-PTX conjugate was synthetized. In this conjugate, PTX is attached by covalent bond to FA and HEP (Figure 3). It was used in experiments on tumor xenografts of human cells cultured subcutaneously or on laboratory animals [125]. This polymeric conjugate can self-assemble into spherical mycelium in the aqueous medium by binding PTX to HEP by hydroxyl grouping and a pH sensitive linker. Cytotoxicity tests have shown that this conjugate possesses significant cytotoxicity against MDA-MB-231 tumor cells, and FA enhances the targeting of the compound [126]. Cell absorption and intracellular distribution was studied by confocal laser scanning microscopy (CLSM) [127].
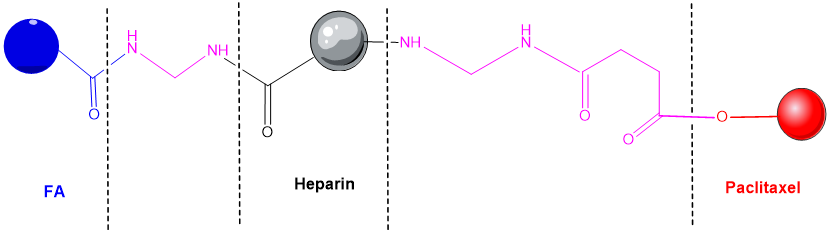
Figure 3. Heparin - folic acid - PTX conjugate [125].
6. Auricularia auricula polysaccharides
A promising drug-delivery system based on a polysaccharide biopolymer was isolated from the medicinal fungus Auricularia auricula. An experimental study concluded that a lectin containing four peptides inhibited the A549 cells proliferation by regulating the expression of some cancer-related genes [128], such as JUN an oncogenic transcription factor [129], TLR4 (toll-like receptor 4) expressed on immune cells and also on tumor cells [130], and MYD88 (myeloid differentiation factor 88) that promotes colorectal cancer cells [131]. NPs containing polysaccharide polymers from Auricularia auricula (AAP) and CTS were very efficient for DOX entrapping and penetrating in tumor cells. Thus, AAP represents a promising option as an antineoplastic drug carrier [132].
7. Protein-drug conjugates and peptide-drug conjugates
7.1. Silk Fibroin
Silk fibroin (SF) produced from the cocoon of Bombyx mori silkworm is widely used and has various biomedical applications, including the controlled release of drugs. Silk fiber derived from other insect species has also been investigated. Silk fibroin consists mainly of fibroin and sericin [133][134][135].
Silk-based delivery systems have excellent properties and can be used to deliver many therapeutic substances for cancer treatment, such as: (i) chemotherapeutics, (ii) nucleic acids, peptides or proteins, (iii) inorganic compounds, (iv) photosensitive molecules, (v) plant derivatives. It has been reported that intracellular degradation of SF NPs is dependent on lysosomal enzymatic function. Therefore, these SF-based nanocarriers perform a critical function, represented by the degradation of the release system, and can be considered a safe in vivo drug delivery systems [134][136].
7.2. Centyrins
Centyrins (CTRs) are non-antibody, small size proteins that are engineered from the human protein Tanascin C (found in extracellular matrix). There are some CTRs’ properties (the lack of disulfide bonds, the small molecular size, increased stability, low immunogenicity, improved tissue penetration, simple drug conjugation, etc) which make these molecules the perfect candidates for targeted delivery applications. CTRs can be conjugated with small interfering ribonucleic acids and an increase in efficiency of gene target modulation was observed [137][138][139].