This substantial blue shift, attributed to complete hydrolysis of phosphorylated probes into insoluble hydroxylated tetraphenylethylene units, resulted in highly ordered aggregates. An ALP-regulated switch on response was found to be highest in the case of ALP-2, followed by ALP-4 and ALP-1, under physiological conditions. In contrast, mono-phosphorylated probe ALP-1 was found to be very sensitive compared to di- and tetra-phosphorylated probes in similar experimental conditions. The scholars speculate that the presence of multiple phosphorylated units in ALP-3 induced a complex mechanism of dephosphorylation with ALP, resulting in low sensitivity. Due to these reasons, even though di-phosphorylated probe showed a higher enhancement ratio upon interaction with ALP, the lowest detection limit was found for the mono-phosphorylated probe. Stoichiometrically, at the specified concentration, the mono-phosphorylated probe has quantitatively half of the di-phosphorylated one’s units. Only mono- and di-phosphorylated probes were analyzed in biological samples because of their higher sensitivity and stability.
Cytotoxicity studies analyzed through propidium iodide revealed that both ALP-1 and ALP-2 can be used effectively at 2 to 20 μM. Upon incubation of 20 μM of probe with ALP-1/2 during osteogenic differentiation in a bone marrow mesenchymal stem cell (BMSC) culture (0 to 7 days), fluorescence intensities were increased in the green channel, and can be visualized through fluorescence confocal laser scanning microscopic images. Overexpression of ALP during osteogenic differentiation of stem cells was further supported through the flow cytometric Western blotting and rt-PCR analysis. It was found that, in cellular conditions, the di-phosphorylated probe ALP-2 showed significant changes compared to that of the mono-phosphorylated counterpart.
Upon exploiting the AIE properties in combination with ESIPT behavior, Tang et al. reported a 2′-hydroxychalcone-based phosphorylated probe
ALP-4 (
Figure 3) for ALP recognition in in vitro and in cellulo
[72]. The rationally designed probe triggered far-red emission centered at 640 nm upon ALP-catalyzed dephosphorylation under physiological conditions.
ALP-4 showed absorption maxima at λ
max 430 and 416 nm in UV–Vis spectra and weak emission (greenish-yellow λ
em 538 nm). This weakly green emissive behavior in the phosphorylated state was attributed to the presence of
N,N,dimethylaniline and vinyl ketone units, thereby creating an ICT process in the chalcone unit in the twisted conformation. However, upon dephosphorylation, the presence of the ketone unit at the
ortho position induced strong intramolecular hydrogen bonding upon photochemical excitation, leading to the highly stable 6-membered ring, resulting in keto-enol tautomerism. Based on pH-dependent study, the scholars speculated that fluorescence properties in the probe should be attributed to the combination of synergistic ESIPT and ICT processes. Single crystal analysis of
ALP-4 (
OH) revealed head-to-head and edge–face stacking resulted in strong intermolecular-coupling-aided AIE behavior. Exploiting such dual photophysical properties in phosphorylated and dephosphorylated 2-hydroxychalcone resulted in ratiometric fluorescence switching properties in green and red channels. The probe detected ALP as scarce as 0.15 mU/mL in a ratiometric manner. The scholars did not specify the kinetic parameters in their work.
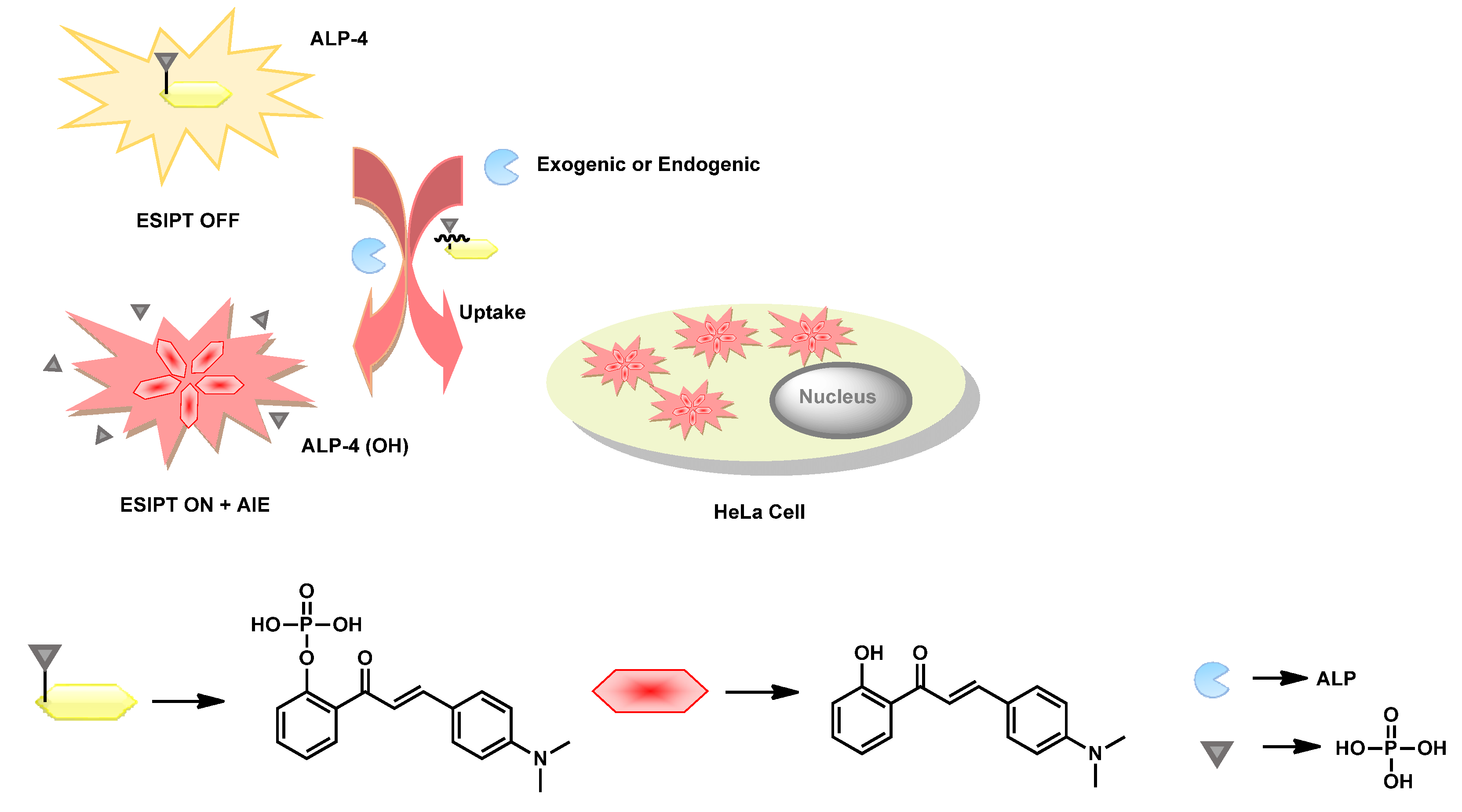
Figure 3. Structure of the ESIPT-AIE-based fluorescent probe ALP-4 and a plausible phosphatase recognition mechanism.
ALP-4 showed excellent biocompatibility towards HeLa cell lines. Upon incubation of ALP-4 in endogenously overexpressed HeLa cells, orange fluorescence was seen in the cytoplasm. The scholars speculated on the existence of unusual blue-shifted emission in the orange region in cellular conditions, as compared to that of in vitro conditions (red emissive), could have been due to the twisted molecular confinements of ALP-4 (OH) upon interactions with bio-macromolecules in biological fluids. To validate the intracellular phosphate hydrolysis of ALP-4, fluorescence confocal imaging studies (FCIS) were performed in dual channels in the absence and presence of the ALP inhibitor levamisole. According to the results, the scholars postulated that intercellular ALPs readily cleaved the phosphorylated probe in extracellular fluid, and thereafter the fluorescent ALP-4 (OH) was transfected passively, resulting in bright fluorescence in the cytosol.
Sun et al. and co-workers reported the naphthalene-based green emissive two-photon florescent probe
ALP-5 (
Figure 4) for in vitro and in cellulo recognition of ALPs
[73]. A PO
43− group was incorporated into the self-cleavable benzyl-ester unit at the ortho position in such a way that, upon dephosphorylation, it could trigger the release of an amino-naphthalene moiety. Under physiological conditions, the probe showed absorption maxima at λ
max 300 nm in UV–Vis spectrum and emission maxima centered at λ
em 450 nm in the fluorescence spectrum. Upon dephosphorylation in the presence of ALP, the absorption and emission maxima shifted to 365 and 500 nm respectively. Such selective bathochromicity in the absorption spectrum, along with a
switch on response in the green channel, made the scholars interested in performing ALP recognitions in biological systems.
Figure 4. Structure of ALP-5 probe and a plausible phosphatase mechanism.
Nie et al. developed a new near-infrared, emissive, hemi-cyanin-based fluorescent probe,
ALP-6 (
Figure 5), for in vitro and in vivo recognition of endogenous ALP activity
[74].
ALP-6 involves phosphorylated chromene units conjugated with benz[e]indolium salts. In buffered conditions, the probe showed an absorption maximum at λ
max at 604 nm and was very weakly emissive (λ
em 738 nm). Upon incubation with ALP at roughly physiological pH, 8.0, there was a 10-fold enhancement (at 738 nm) in the emission intensity, along with a substantial redshift from 604 to 738 nm in the UV–Vis spectra within 20 min. The designed hemicyanine-based probe showed a highly selective and robust
switch on response in the NIR region, towards phosphatase, without allowing any interference by biologically relevant ions or macromolecules. The ALP-induced dephosphorylation of
ALP-6 retained a strong ICT process, resulting in high fluorescence with redshifts in the UV–Vis spectrum, which were significantly inhibited by the phosphorylated probe due to the electron pulling effects from the electronegative phosphorous atom in the PO
43− unit.
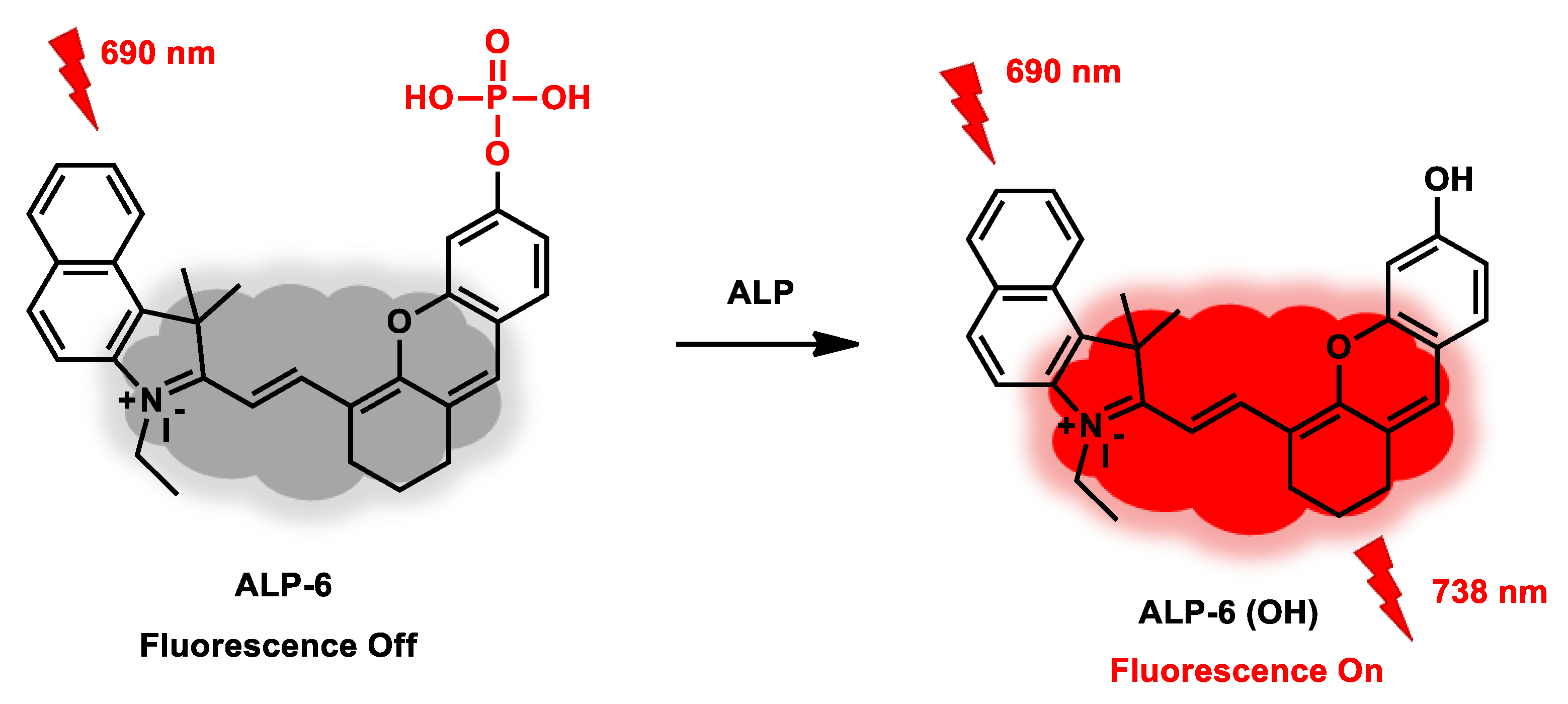
Figure 5. Structure and a plausible phosphatase recognition mechanism of probe ALP-6.
Liang’s group reported fluorescent peptide
ALP-7 (Figure 6), for the in vitro recognition of ALP. The designed FITC-conjugated, phosphorylated peptide undergoes dephosphorylation in the presence of ALP, leading to the three-dimensional, self-assembled, fibrous-type material—a “gel”
[75] at pH 8.0 (TRIS-HCl). Enzyme-assisted sol to gel transformation resulted in quenching of fluorescence in the green channel attributed to conventional aggregation caused quenching (ACQ). The scholars did not specify kinetic parameters such as
Vmax and
Km.
ALP-7 showed strong green emission upon illumination at 365 nm (UV light), whereas upon incubation with ALP, in physiological pH buffers, a substantial decrement in emission intensity in the green channel was observed. Concomitantly, a significant color change from light green to transparent yellow was clearly seen with the naked eye. Probe was able to recognize ALP as lowest as 0.06 U/mL in similar experimental conditions.
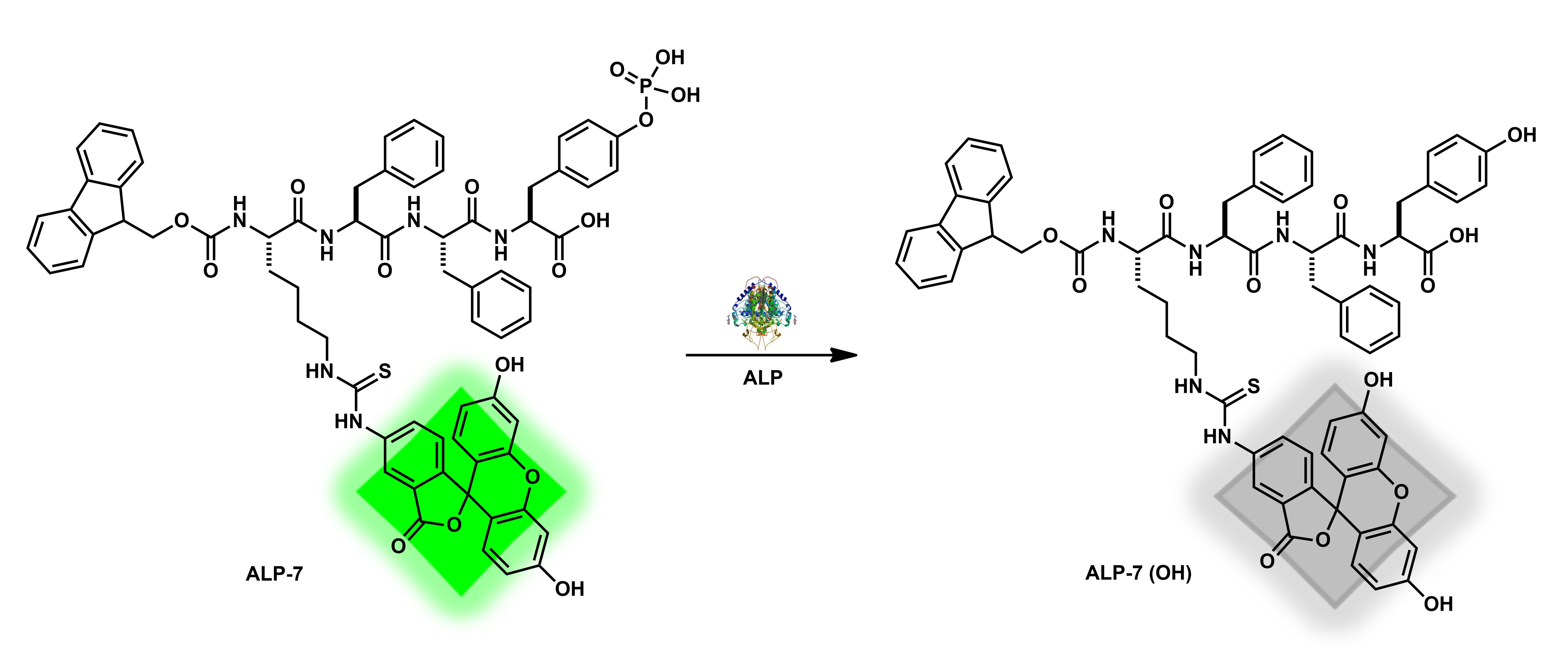
Figure 6. Structure and a plausible phosphatase recognition mechanism of probe ALP-7.
Tan et al. reported a hemicyanine-based NIR based fluorescent probe,
ALP-8 (
Figure 7), for in vitro and in vivo recognition of ALP
[76]. In buffered conditions, the probe showed absorption maxima at λ
max 600 and 650 nm in the UV–Vis spectrum, and did not induce any fluorescence band in the NIR region. Upon dephosphorylation, absorption maxima λ
max were red-shifted to 650 and 680 nm, elicited with bright NIR fluorescence centered at 700 nm. They observed blue shifts in the absorption and emission wavelengths compared to
ALP-6 (benz[e]-indolium core), revealing that indolyl core’s conjugation extension can significantly affect the ICT process in hemicyanine-based fluorophore units.
Figure 7. Structure of hemicyanine-based probe ALP-8 and a plausible phosphatase recognition mechanism.
Wei et al. reported a highly efficient, NIR-regulated far-red probe,
ALP-9 (
Figure 8) based on the dicyanomethylene-4H-chromene unit conjugated with dichrolorophenyl phosphate ester
[77]. To achieve best possible tumor microenvironment (TME) localization and controlled time-resolved intracellular ALP imaging,
ALP-9 was loaded into am NIR-responsive nano-container.
Figure 8. The structure of the NIR-regulated far-red emissive probe ALP-9 and a plausible phosphatase recognition mechanism in the TME.
Ding et al. reported a novel D–π–A-tuned mitochondria-targeting fluorescence probe (
ALP-10) whose emission properties are regulated through the ESIPT phenomenon
[78]. Molecular engineering was performed by the combination of 2-(2′-hydroxyphenyl)-benzothiazole (ESIPT core) with a 4-styryl-pyridinium (robust mitochondrial targeting unit) core (
Figure 9).
ALP-10 showed a characteristic broad absorption peak with maximal absorptivity at ca. ~358 nm and an emission maximum at 514 nm (ϕ = 0.21) in UV–Vis and fluorescence spectra, respectively. Upon ALP induced dephosphorylation, the free phenolate unit induced a strong ESIPT process in
ALP-10 (
OH), resulting in the generation of a new absorption band (λ
max ca. ~510 nm) and emission band (λ
em ca. ~650 nm). The dephosphorylated product with free phenolic OH emitted a strong red fluorescent signal in a ratiometric manner. The ALP recognition capability of the probe was found to be appreciable in the physiological pH range (5.0 to 8.0). However, at an alkaline pH (pH > 8.0) the ratiometric ALP recognition capability was significantly diminished, which was attributed to inhibition of the ESIPT process pertaining to phenolate ion formation.
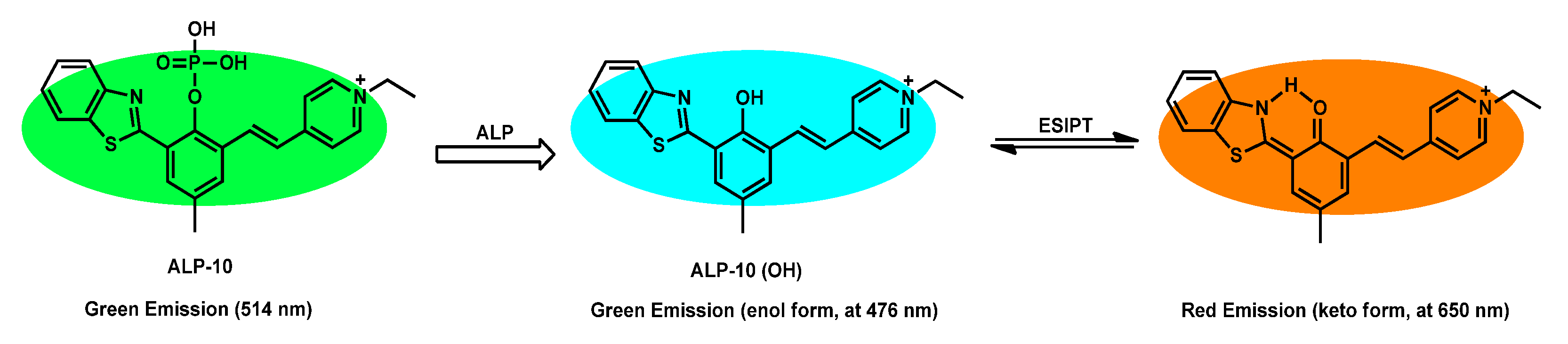
Figure 9. Structure of ALP-10 and its plausible sensing mechanism for phosphatase.
Han et al. reported the 2′-(2′-hydroxyphenyl)-benzothiazole (HBT) fluorescent conjugates (
Figure 10) were linked to the 5′ position of the ribonucleoside (adenosine/guanosine) residues for recognition of ALP in cellular conditions
[79]. These 5′-ribonucleotide-fluorophore analogues showed weak emission, due to the phosphorylated phenolic OH in HBT unit. P–O bond cleavage (dephosphorylation) generates free HBT and phenolic OH, which exists in a keto–enol tautomerization equilibration. Upon photochemical excitation, the ESIPT core equilibrium shifted toward the enol tautomer (more predominant isomer), resulting in red shifts in the emission spectrum.
ALP-11/12 are non-fluorescent in aqueous buffer, but otherwise have three emission maxima, 370, 375 and 380 nm. Similarly, these probes exhibited a bright fluorescent signal centered at 512 nm in the presence of ALP.
Figure 10. Structures of phosphatase probes ALP-11 and ALP-12. (In ALP-11; R1 = NH2, R2 = H, ALP-12; R1 = O, R2 = NH2).
Kim et al. reported a new iminocoumarin-benzothiazole-based fluorophore,
ALP-13 (
Figure 11) to recognize the phosphatase at the single-cell level
[80]. In an aqueous buffer solution, probe showed an absorption maximum of 472 nm and emission maximum (λ
em) of 542 nm, in the UV–Vis and fluorescence spectra, respectively.
Figure 11. Structure and phosphatase recognition mechanism of iminocoumarin-benzothiazole-based probe ALP-13.
Li et al. reported the self-immolative resorufin-based probe
ALP-14 (
Figure 12) for an ALP assay in cellular conditions
[81]. Molecular engineering was performed based on the conjugation of an ALP-recognizing substrate (phosphoester unit) to a red emissive resorufin unit through the reactive
p-hydroxybenzyl unit, which acts as self-immolative linker. This design is such that ALP can easily access the phosphor-ester terminus of the probe without steric hindrance, for biocatalytic dephosphorylation. When
ALP-14 is dephosphorylated, it intermediately undergoes 1,6-eliminations to produce a
p-quinone methide ether derivative and a free
Resorufin unit. This transformation is associated with changing of the solution’s color from orange to purple and generating intense fluorescence emission centered at the 585 nm region.
Figure 12. Structure of the self-immolative resorufin-based chromo-fluorescent probe ALP-14 and a plausible phosphatase recognition mechanism.
Sun et al. reported a novel hydrazone-based fluorescent probe,
ALP-15 (
Figure 13), for the in cellulo recognition of ALP. The opto-analytical behavior of the probe is regulated through the dual photophysical phenomena, such as aggregation-induced emission (AIE) and excited state intramolecular proton transfer (ESIPT)
[82]. The design strategy targeted a simple and small molecular architecture, which showed excellent selectivity and sensitivity, by showing a large Stokes shift upon interacting with phosphatase in physiological conditions.
Figure 13. Structure of ALP-15 and its plausible recognition mechanism with ALP.
Wu et al. developed a 1,8-naphthalimide derivative,
ALP-16, for real time ratiometric recognition of (
Figure 14) the organ damage biomarker ALP in cells and in vivo models
[83]. A phosphor-ester group was incorporated at the 4th position of the 1,8-naphthalimide core to induce dual emission (blue–green region) and incorporation of a peripheral amine-
N-oxide group facilitated water solubility and biocompatibility. Upon phosphor-ester hydrolysis, the 4th position of the naphthalimide unit is transformed into free OH. This chemical transformation enormously changes the photophysical properties of the probe, causing a dramatic color change and a ratiometric signal in the emission spectra. In the biological buffer,
ALP-16 exhibited an absorption maximum λ
max at ca. ~375 nm, and an emission maximum at λ
em 468 nm in the UV–Vis and fluorescence spectra, respectively. Upon ALP-regulated biocatalytic dephosphorylation, electron deficient substituents (phosphoester) were changed to electron donating substituents (phenolic OH) at the 4th position of the 1,8-naphthalimide unit of
ALP-15 (OH). Due to these reasons, significant bathochromic behavior (λ
max 375 → 450 nm) was observed in UV–Vis spectra. Similarly, significant ratiometric changes were observed through the concomitant decrement at 468 nm and a significant enhancement at 554 nm (redshift) in the emission signal.
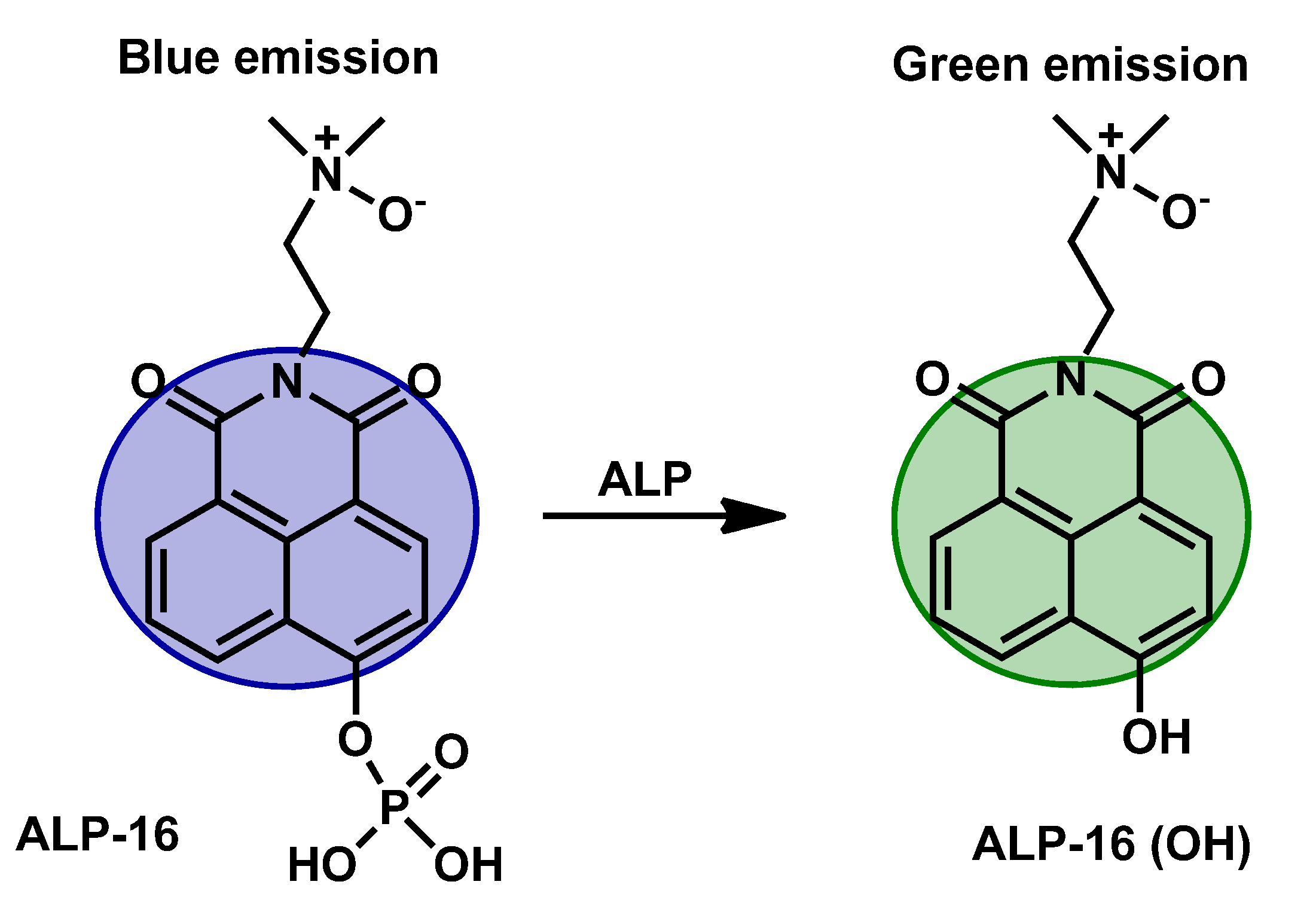
Figure 14. The structure of ALP-16 and a plausible phosphatase recognition mechanism.
Zhou et al. reported a naphthalene-based two-photon fluorescent probe
ALP-17[84] for the recognition of ALP (
Figure 15) in a ratiometric manner. The probe’s fluorescence intensity ratio showed a linear relationship with ALP concentration in the range of 20 to 180 U/L, and had a detection limit of 2.3 UL
−1 in physiological conditions. The scholars did not specify the dephosphorylation kinetic parameters in their work. In a detailed in vitro investigation,
ALP-17 was used to recognize the intracellular ALP activity using one-photon (405 nm) or two-photon (720 nm) excitation techniques. The probe’s quantum yield was found to be 0.41. When excited at 720 nm, the probe has a 65 GM two-photon action absorption cross-section at 428 nm. The probe’s cytotoxicity was evaluated based on MTT assay in HeLa cells, revealing that
ALP-17 did not have a toxic effect until 20 µM. During incubation with
ALP-17, HeLa cells exhibited bright green fluorescence after 30 min. In contrast, probe incubated in levamisole-hydrochloride-pre-treated HeLa cells resulted in only blue emission, which supports that
ALP-17 is able to detect intracellular ALP. The probe-incubated HeLa cells resulted in bright fluorescence images upon excitation at λ
ex 720 nm, supporting the idea that
ALP-17 is suitable for two-photon imaging as well.
Figure 15. The structure of
ALP-17 and a plausible phosphatase recognition mechanism.
Podder et al. developed the rhodol-based green fluorescent probe
ALP-18 (
Figure 16), which was used to distinguish cancer cells from normal cells based on lysosomal phosphatase expression
[85]. In vitro studies revealed that the probe causes a ~9-fold increment in UV-absorption at λ
abs of 490 nm and a ~33-fold emission enhancement at λ
em 532 nm in the presence of 1.72 U mL
−1 of ALP. In this probe, fluorescence emission behavior was tuned through the closed and open structure of the conventional spiro-lactam ring in the rhodol skeleton. In the phosphorylated state, the probe exists in spirocyclic form and exhibited weak fluorescence. However, upon dephosphorylation, the spiro-lactam ring will be opened, resulting in revival of rhodol fluorescence in the green channel. Dephosphorylation kinetic parameters
KM, k
cat and k
cat/
KM were found to be 7.0 µM, 9.52 s
−1, and 13.6 × 10
5 M
−1 s
−1, respectively. The probe did not suffer from interference in the presence of biologically relevant ions and molecules in physiological conditions.
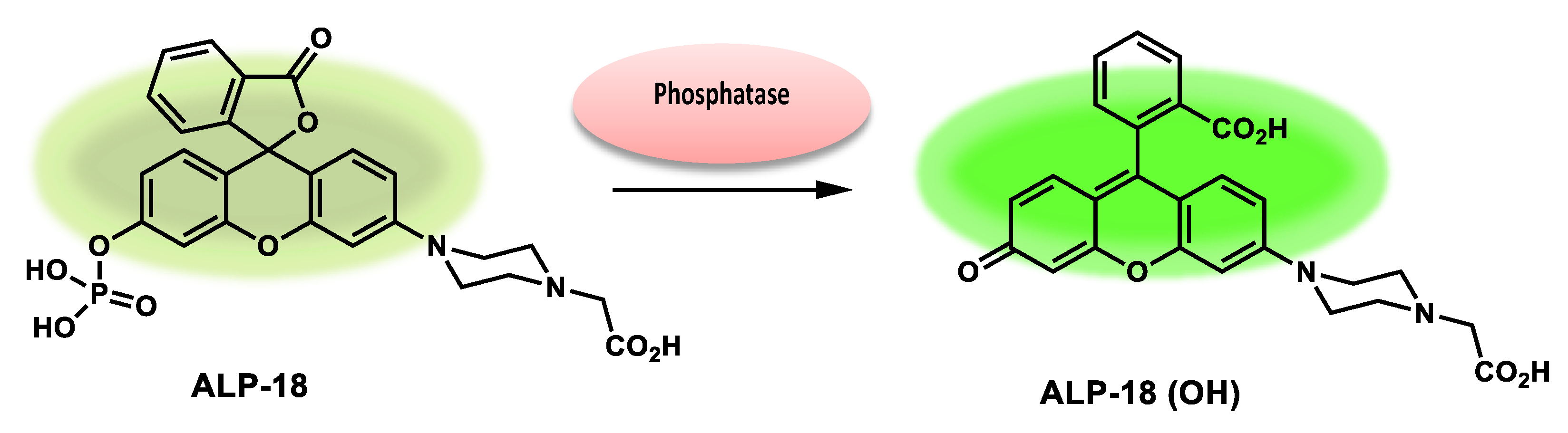
Figure 16. Structure of ALP-18 and a plausible phosphatase recognition mechanism.
Lu et al. reported ICT tuned dicyano-based fluorogenic substrate (
ALP-19) for the ratiometric recognition of ALP (
Figure 17)
[86]. The probe showed a significant bathochromic shift (550 → 650 nm) in the emission spectrum, upon biocatalytic dephosphorylation: a substantial color change from yellow-green to light orange. In its phosphorylated state, the probe was weakly emissive due to lowering of electron densities on the phenolic oxygen, which resulted in inhibition of the ICT phenomenon in D–π–A skeleton. However, upon phosphate hydrolysis, phenolic OH enhanced the ICT phenomenon in the probe, resulting in noticeable spectral shifts in the UV–Vis and emission signals. The absorption and emission bands on the probe
ALP-19 exhibited peaks at 440 nm (ε = 2.2 × 10
4 M
−1 cm
−1) and 550 nm (ϕ = 10.5%) respectively. Upon dephosphorylation, a new band appeared at 650 nm (ϕ = 7.5%). It is worth noting that due to the weak emission at 650 nm, the dephosphorylated probe exhibited a low quantum yield compared to the phosphorylated form.
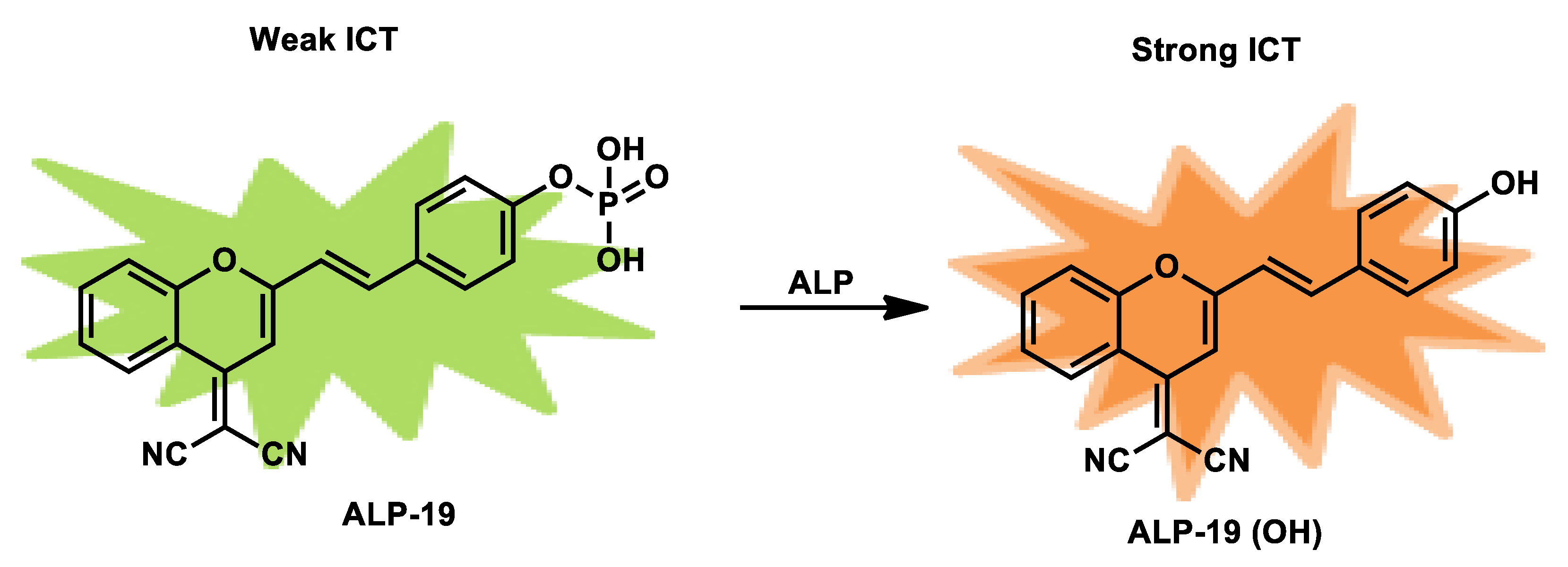
Figure 17. Structure of the far-red emissive dicyano-based fluorescent probe ALP-19 and a plausible phosphatase recognition mechanism.
Hu et al. reported a new flavone-based fluorescent probe,
ALP-20 (
Figure 18) (3-hydroxy-2-(p-tolyl)-4H-chromen-4-one), for the in vitro and in cellulo recognition of ALP
[87]. Tuning of photophysical behavior in the probe is attributed to inhibition and facilitation of the ESIPT process in phosphorylated and dephosphorylated states, respectively. Due to the presence of a phosphorylated phenolic unit, it is unable to undergo a tautomerization process; as a result, emission intensity is significantly quenched. However, ALP-regulated dephosphorylation induced the keto-enol tautomers through the phenolic OH group, allowing for intensified ESIPT-based emission.
Figure 18. Structure of the flavone-based fluorescent probe ALP-20 and a plausible phosphatase recognition mechanism.
Liu et al. reported a hemicyanine-based NIR fluorescent probe,
ALP-21 (
Figure 19), for turn-on trapping of ALP activity in cancer cells and tumor-xenografted nude mouse models
[88]. The probe rationale was based on regulation of the intramolecular charge transfer (ICT) principle. The phosphate group was tethered directly to the fluorophore’s phenolic OH group, thereby preventing fluorescence in the hemicyanine core by repressing the ICT process. Upon biocatalytic dephosphorylation, free phenolic OH was regenerated, thereby reviving a strong ICT process which triggered a bright emission signal in the NIR region.
Figure 19. Structure and a plausible phosphatase recognition mechanism of the NIR fluorescent probe ALP-21.
Li et al. reported
ALP-22, a water-soluble hemicyanine-based NIR fluorescent probe (
Figure 20) tethered with a quinolinium ethyl iodide unit to induce water solubility
[89].
ALP-22 showed absorption maxima at λ
max 568 and 720 nm in UV–Vis spectrum, before and after addition of phosphatase, respectively. Substantial changes in absorption maxima involved a dramatic color transition from purple to blue. The phosphorylated probe was weakly emissive due to the inhibition of ICT by the phosphoester unit. Upon dephosphorylation, a strong ICT process was resurrected between the quinolinium unit and the chromene-type phenolic unit, resulting in bright emission centered at 770 nm (~7 fold).
ALP-22 showed excellent selectivity and sensitivity towards ALP in vitro, allowing it to be used to visualize ALP activity in various cells.
Figure 20. Structure of the quinolinium-based NIR fluorescent probe ALP-22 and a plausible phosphatase recognition mechanism.
Zhang et al. reported a far-red fluorescent probe based on malononitrile tethered chromene analogue
ALP-23 (
Figure 21) for in vitro and in vivo detection of ALP
[90]. The design rationale of
ALP-23 was based on the phosphorylation and dephosphorylation of the phenolic OH unit, accomplished through the inhibiting and restraining of the ICT process in D–π–A confinements. The ALP-regulated dephosphorylation reaction restores the ICT effect by unveiling the “turn-on” fluorescence in the
ALP-23 (
OH). The probe showed absorption spectra ranging from 420 to 580 nm with a sharp absorption maximum at 510 nm and annihilated red emission upon excitation at 600 nm. However, upon addition of ALP, the absorption band at 510 nm was decreased and saw a substantial shift to 605 nm. Additionally, a prominent emission signal was observed with maximal intensity at 640 nm. Such substantial changes in optical behavior in
ALP-23 were attributed to biocatalytic dephosphorylation, via the strong ICT process in
ALP-23 (
OH).
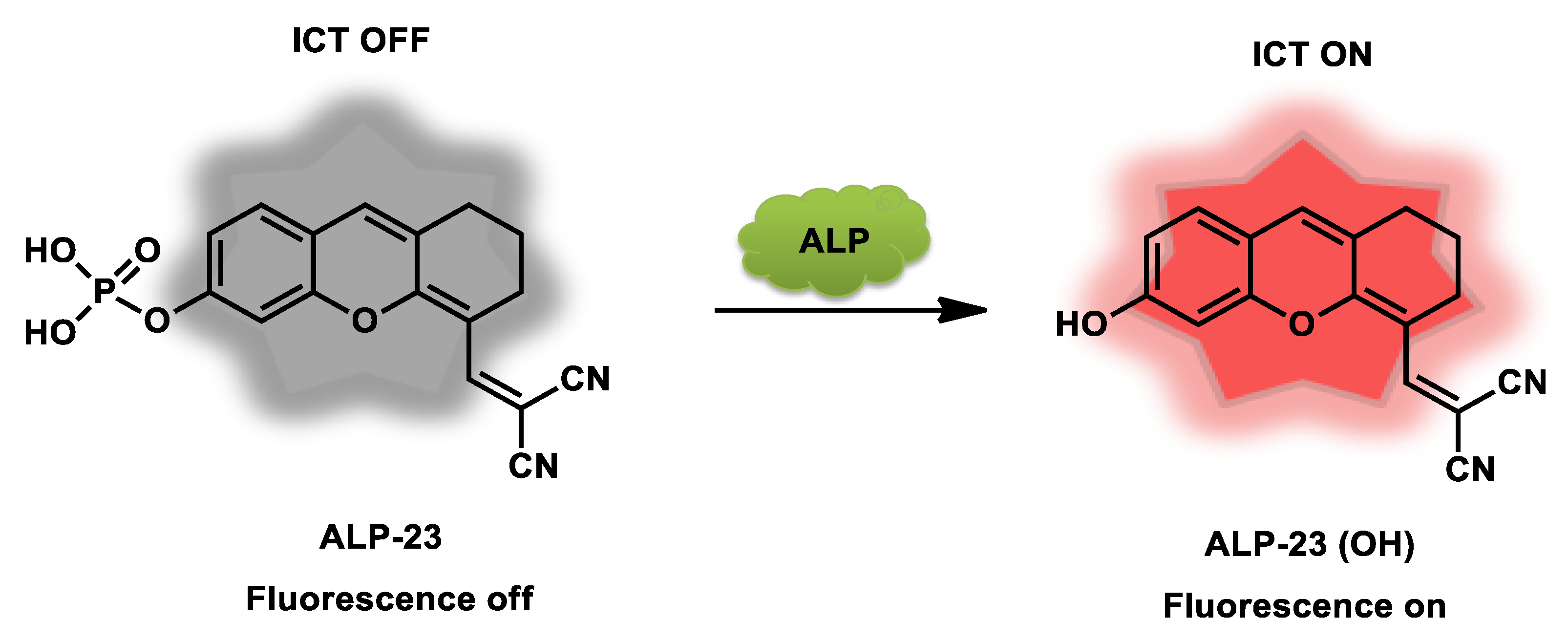
Figure 21. Structure of the di-cyanovinylene-based far-red fluorescent probe ALP-23 and a plausible phosphatase recognition mechanism.
Khatun et al. reported coumarin-tethered triphenylphosphonium salt
ALP-24 for the recognition of (
Figure 22) mitochondrial ALP in cellular conditions
[91]. The rationale was based on a receptor-fluorophore-subcellular targeting group where coumarin acts as a signaling unit (blue fluorophore), in which the ALP receptor (diethyl phosphate) is attached to the 8th position, and the mitochondrial targeting moiety triphenylphosphine is connected to a coumarin unit through the ethyl spacer. The diethyl-phosphate moiety was retained in the molecule to enhance the cellular uptake, instead of the conventional negatively charged free phosphate terminus.
Figure 22. Structure of the mitochondria-targeting fluorescent probe ALP-24 and a plausible phosphatase recognition mechanism.
Park et al. reported the two dihydroxanthene-based NIR fluorescent probes
ALP-25 and “
ALP-26” (
Figure 23) for the detection of ALP in cells and tissues
[92]. To induce better water solubility, phenolic and sulfonate groups were incorporated at each terminus. The rationally designed probes were non-fluorescent in their phosphorylated states; however, upon biocatalytic dephosphorylation, the probes exhibited bright NIR fluorescence, in a dose-dependent manner. ALP-regulated
off–on fluorescence switching in the probes was attributed to inhibition and restoration of the ICT process in the hemicyanine core.
ALP-25 and
ALP-26, due to the minor difference in the hemicyanine core substitution, both showed a characteristic electronic absorption band around 600–700 nm in their UV–Vis absorption spectra. Both probes were weakly emissive (Φ
F = ~0.006) due to the effective inhibition of the ICT process. However, in the presence of ALP, a new band was generated at ~710 nm with a substantial increment in NIR emission intensity (~200 fold, Φ
F = ~0.009), upon excitation at 685 nm. The probes showed a rapid
switch on response towards ALP (0.1 U min
−1) within 1.5 min. However, the scholars did not report any kinetic parameters, such as
Km and
Vmax, in their work.
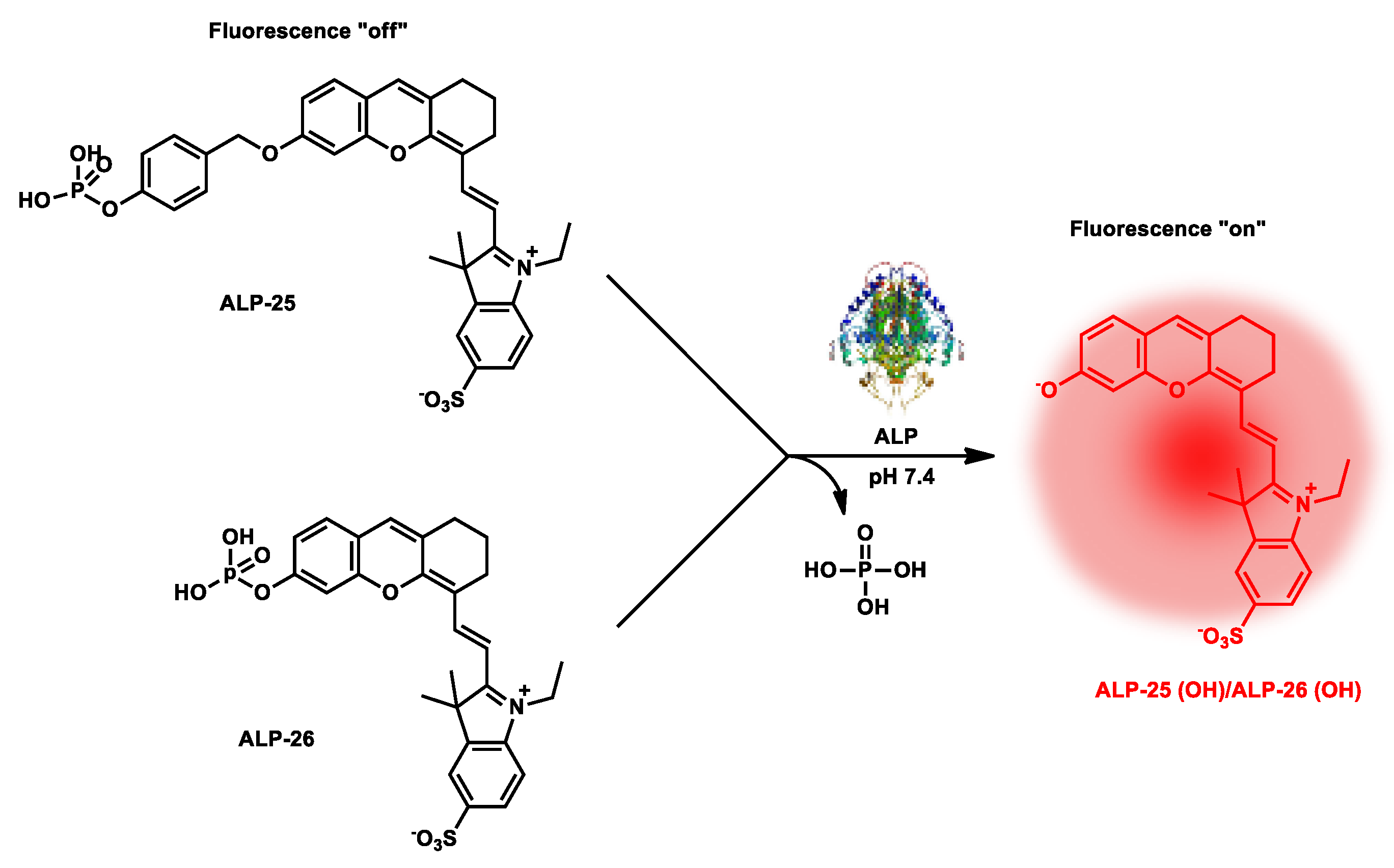
Figure 23. Structures of NIR fluorescent probes ALP-25 and ALP-26 and a plausible phosphatase recognition mechanism.
Upon detailed in vitro analysis, the probes’ (ALP-25 and ALP-26) binding affinities towards hydroxyapatite (HA) were studied in the presence of various types of calcium salts, such as phosphates, carbonates, chlorides and nitrates. Intriguingly, they showed high binding affinities towards the HA surface. The scholars believed that the phosphate unit in the probes being able to interact with the Ca2+ ions on the HA surface is the main reason for their superior adsorption. It was found that the probe-holding calcium phosphates scaffolds have moderately good ALP recognition capabilities in physiological conditions.
Yaqian et al. reported hydrazone-based AIE-tuned fluorescent probe €-2-(((9H-fluoren-9-ylidene) hydrazono)methyl)phenyl dihydrogen phosphate,
ALP-27, for the recognition of cellular ALP
[93]. The probe’s rationale is based on the dual photophysical phenomena ESIPT and AIE.
ALP-27 exhibited a characteristic absorption maximum at 400 nm and was weakly emissive upon excitation at λ
ex 380 nm in physiological conditions. However, upon biocatalytic dephosphorylation, the probe showed bright emission centered at 586 nm by showing minor changes (blue shift 380 →350 nm) in the UV–Vis absorption spectra (
Figure 24). ALP-regulated dramatic green emissive behavior exhibited a large Stokes shift (>200 nm), which was attributed to the synergistic AIE and ESIPT mechanism.
Figure 24. The structure and a plausible phosphatase recognition mechanism of the probe ALP-27.
Yin et al. reported a novel red emissive fluorescent probe,
ALP-28 (
Figure 25), based on isophorone for intracellular ALP detection
[94]. In physiological conditions, the probe showed an absorption maximum at 404 nm and weak emission centered at 570 nm in UV–Vis and fluorescence spectra, respectively. Upon phosphatase catalyzed hydrolysis, the probe exhibited substantial bathochromicity (404 → 421 nm) in the UV–Vis spectrum and an intense orange–yellow fluorescence signal centered at ca. 570 nm in the emission spectrum. The probe showed excellent sensitivity towards ALP with a detection limit of 0.088 U/L in in vitro assay conditions. Biocatalytic dephosphorylation of
ALP-28 was authentically validated through the
31P NMR spectroscopic methods. In the studies before and after dephosphorylation, the phosphorous resonance peak was at δ −4.56 ppm or δ 0.81 ppm, respectively, unambiguously supporting the ALP-regulated phosphoester hydrolysis.
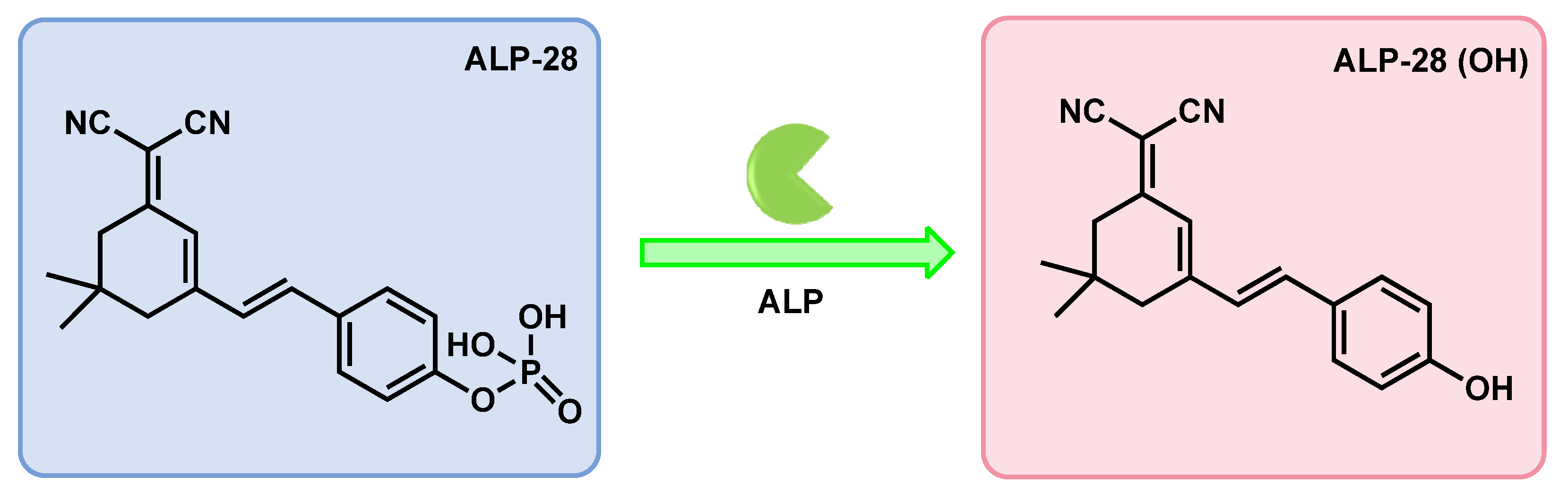
Figure 25. Structure and a plausible phosphatase recognition mechanism of the probe ALP-28.
Yangyang et al. reported a benzothiazole-based ratiometric probe, (4-benzamio-2-(benzo[d] thiazol-2-yl)phenyl dihydrogen phosphate (
ALP-29), for the intracellular recognition of ALP
[95]. Its molecular engineering associated it with the phosphatase-regulated ESIPT phenomenon (
Figure 26). In order to reduce the pH-related instability in ESIPT-based probes, the phenolic‘s unit para position was functionalized with a benzoamide moiety. Under physiological conditions, the probe showed characteristic excitation and fluorescence emission peaks at ca. 340 and ca. 425 nm respectively. Upon biocatalytic dephosphorylation, bright red-shifted (120 nm) fluorescence emission was observed (425 nm → 545 nm). Such dramatic changes in fluorescence intensity were attributed to revival of the ESIPT mechanism, resulting from the phenolic OH unit with a benzothiazole nitrogen forming the partial quinone structure upon photochemical excitation.
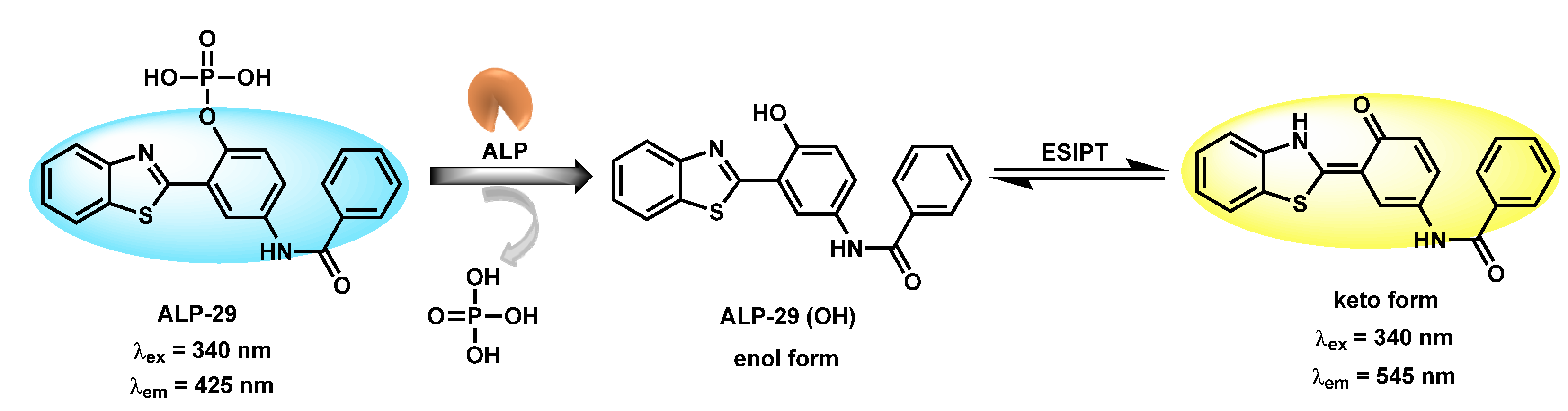
Figure 26. Structure and plausible phosphatase recognition of probe ALP-29.
Gao et al. reported a new blue–green emissive fluorescent probe,
ALP-30 (
Figure 27), based on a naphthalimide core, for in cellulo ratiometric imaging of ALP
[96]. In physiological conditions,
ALP-30 exhibited an absorption maximum at λ
abs ca. 355 nm and itself has an emission maximum at ca. 472 nm, due to the weak electron withdrawing effect (weak ICT) of its phosphoester group. Upon phosphatase assisted cleavage of the phosphoester unit, there was a new absorption maximum at λ
abs ca. 448 nm and a new emission peak at 556 nm, owing to the strong electron donating ability of -OH (strong ICT). Strong green emissive behavior in the dephosphorylated probe (
ALP-30 (
OH)) can be attributed to the formation of 4-hydroxyl-naphthalimide, which resulted in the generation of the ICT process with the electron donating (resonance stabilized) phenolic OH and the electron accepting imide core.
Figure 27. Structure and a plausible phosphatase recognition mechanism of ALP-30.
Wang et al. reported a novel hemicyanine-based water-soluble NIR fluorophore
ALP-31 (
OH) (
Figure 28) (3-ethyl-2-(2-(6-hydroxy-2,3-dihydro-1H-xanthen-4-yl)vinyl)benzo[d]thiazol-3-ium) for exogenous and endogenous ALP imaging in cells
[97]. The probe’s rationale revolved around a simple push–pull tuned ICT process between the phosphorylated/dephosphorylated phenolic OH and the benzothiazolium unit. The phosphorylated state’s ICT process is inhibited due to the electron withdrawing capability of the phosphoester unit; as a result, the probe was weakly emissive (ϕ = 0.15). However, dephosphorylated
ALP-31 (
OH) exhibited a strong fluorescence signal with an excitation maximum at ca. 680 nm and an emission maximum at ca. 723 nm. The strong ICT process between the phenolic OH and thiazolium units culminated in a Stokes’ shift of 43 nm.
Figure 28. Structure and a plausible phosphatase recognition mechanism of ALP-31.
Wu et al. reported an AIE-responsive tetraphenylenthene fluorescent probe,
ALP-32 (
Figure 29), for the recognition of ALP in living cells
[98]. The probe’s rationale is associated with the incorporation of a hydrophilic polar phosphate terminus—a specific receptor for ALP with a hydrophobic TPE core. In biological buffers, the probe showed a characteristic absorption maximum at λ
max ca. 375 nm and extremely weak emissive behavior. Upon dephosphorylation, due to the elimination and formation of polar phosphate and phenol groups, respectively,
ALP-32 (
OH) resulted in bright green emission. These biochemically catalytic transformations led to characteristic absorption at λ
max ca. 400 nm and a ~12-fold enhancement in emission intensity at λ
em ca. 545 nm. Elevated hydrophobicity in
ALP-32 (
OH) resulted in poor water solubility and strong green fluorescence upon aggregation through the AIE mechanism.
Figure 29. Structure and a plausible phosphatase recognition mechanism of probe ALP-32.
Table 1. List of the small phosphorylated molecular probes for ALP activity in cells/tissues/in vivo models.
Probes |
Linear Range |
kcat/KM Value |
Detection Limit |
Response Time |
Stokes Shift |
λex/λem (nm) |
Quantum Yield (Φ) |
IC50 Value |
Sensing Mode |
Application |
Cytotoxicity |
Cellular Localization |
Ref. |
ALP-1, ALP-2, ALP-3 |
10–50 mU/mL, 10–40 mU/mL |
/ |
/ |
/ |
/ |
338/469, 337/457, 337/469 |
0.075, 0.118, 0.101 |
/ |
Turn-on Fluorescence |
Cellular imaging in living stem cells |
30 µM |
Bone marrow mesenchymal stem cells |
[71] |
ALP-4 |
0–150 U/L |
/ |
0.15 U/L |
/ |
211 nm |
430/539, 641 |
/ |
/ |
Ratiometric |
Live cell imaging |
20 µM |
Intercellular fluids |
[72] |
ALP-5 |
0.5–10 U/L |
/ |
0.30 U/L |
/ |
135 nm |
365/500 |
/ |
7.39 µM |
Turn-on Fluorescence |
In living cells and tissues |
20 µM |
Cellular and tissue lysates, cytoplasm |
[73] |
ALP-6 |
0.01–2.0 U/mL |
/ |
0.003 U/mL |
20 min |
/ |
690/738 |
/ |
141.9 µM |
Turn-on Near-Infrared Fluorescence |
In Vivo Cell, tissue and living animal |
30.0 µM |
/ |
[74] |
ALP-7 |
0–2.8 U/mL |
/ |
0.06 U/mL |
2 h |
/ |
465/530 |
/ |
/ |
Turn-Off Fluorescence |
in Vitro and in Living Cells |
160 µM |
/ |
[75] |
ALP-8 |
0–0.1 U/mL |
1.01 × 105 M−1 s −1 |
0.07 U/L |
2 min |
/ |
680/700 |
/ |
7.51 µM |
Off−On Near-infrared Fluorescence |
Living Cells and Mice |
20 µM |
/ |
[76] |
ALP-9 |
0.5–8.0 U/L |
/ |
0.072 U/L |
/ |
/ |
535/683 |
/ |
/ |
Rational Turn-on Red-Near-Infrared fluorescence |
In vivo and in vitro |
50 µM |
Mitochondria, Lysosome |
[77] |
ALP-10 |
0–60 mU/mL |
/ |
0.072 mU/mL |
/ |
260 nm |
390/476, 650 |
0.16 |
10 µM |
Ratiometric |
living cells and in vivo |
10 µM |
Mitochondria |
[78] |
ALP-11, ALP-12 |
/ |
/ |
/ |
/ |
/ |
405/500–530 |
/ |
10 µM |
Turn-on Fluorescence |
Living cells and zebrafish model |
10 µM |
/ |
[79] |
ALP-13 |
/ |
1.4 × 104 M−1 s −1 |
/ |
/ |
/ |
472/542 |
0.002 |
36 µM |
Turn-on Fluorescence |
Living cells |
10 µM |
/ |
[80] |
ALP-14 |
/ |
1.7 × 104 M−1 s −1 |
1.09 U/L |
/ |
/ |
550/585 |
0.0023 |
7.58 µM |
Turn-on Fluorescence |
Living cells |
/ |
Cytosol |
[81] |
ALP-15 |
0–10 U/L |
/ |
0.012 U/L |
/ |
180 nm |
356/536 |
/ |
/ |
Turn-on Fluorescence |
Living cells |
/ |
/ |
[82] |
ALP-16 |
/ |
/ |
0.38 U/L |
/ |
/ |
425/554 |
/ |
/ |
Ratiometric |
In vivo |
5 µM |
/ |
[83] |
ALP-17 |
20–180 U/L |
/ |
2.3 U/L |
/ |
/ |
405/410–460, 470–530 |
0.41 |
/ |
Ratiometric |
Living cells |
20 µM |
/ |
[84] |
ALP-18 |
/ |
13.6 × 105 M−1 s −1 |
0.011 U/L |
/ |
/ |
490/540 |
0.0487 |
/ |
Turn-on Fluorescence |
In vitro, cell imaging and cell cultures |
100.0 µM |
Lysosome |
[85] |
ALP-19 |
50–200 U/L |
/ |
3.8 U/L |
30 min |
/ |
440/550 |
0.105 |
/ |
Ratiometric |
Living cells |
10 µM |
/ |
[86] |
ALP-20 |
/ |
/ |
0.032 U/L |
/ |
/ |
400–410/455 |
0.085 |
/ |
Turn-on Fluorescence |
Live cells and serum samples |
50 µM |
/ |
[87] |
ALP-21 |
1–30 U/L |
/ |
0.28 U/L |
/ |
/ |
680/690–800 |
/ |
/ |
Turn-on Fluorescence |
In vivo and in vitro |
20 µM |
/ |
[88] |
ALP-22 |
0.05–1.0 U mL−1 |
/ |
0.017 U mL−1 |
/ |
/ |
720/770 |
/ |
109.6 µM |
Turn-on Fluorescence |
Cell imaging, treatment of diabetes |
30 µM |
/ |
[89] |
ALP-23 |
5–100 U/L |
/ |
0.28 U/L |
/ |
/ |
600/620–850 |
/ |
141.4 µM |
Turn-on Fluorescence |
Living cells and zebrafish |
10 µM |
/ |
[90] |
ALP-24 |
/ |
3.12 × 106 M−1 s −1 |
2.921 ng/mL |
/ |
/ |
410/450 |
0.567 |
/ |
Ratiometric |
Live cells |
50 µM |
Mitochondria |
[91] |
ALP-25, ALP-26 |
0–1.0 U mL−1 |
/ |
10−5–10−3 U mL−1 |
1.5 min |
/ |
685/710 |
0.13 |
/ |
Turn-on fluorescence |
In vivo |
50 µM |
/ |
[92] |
ALP-27 |
1–100 U/L |
/ |
0.6 U/L |
/ |
>200 nm |
380/586 |
/ |
/ |
Turn-on fluorescence |
Living cells |
10 µM |
Lipid droplets |
[93] |
ALP-28 |
/ |
/ |
0.088 U/L |
6 min |
/ |
440/570 |
/ |
/ |
Turn-on Fluorescence |
Living cells |
10 µM |
/ |
[94] |
ALP-29 |
/ |
/ |
0.004 mU/mL |
35 min |
/ |
340/545 |
0.028 |
/ |
Ratiometric |
Living cells |
20 µM |
/ |
[95] |
ALP-30 |
0–200 U/L |
/ |
0.25 U/L |
/ |
/ |
425/472 |
/ |
/ |
Ratiometric |
Living cells |
/ |
/ |
[96] |
ALP-31 |
0–8 U/L |
/ |
0.042 U/L |
/ |
43 nm |
680/723 |
0.15 |
23.98 µM |
Turn-on Near-Infrared Fluorescence |
Living cells |
20 µM |
/ |
[97] |
ALP-32 |
/ |
/ |
14.2 U/L |
/ |
/ |
440/543 |
/ |
/ |
Turn-on Fluorescence |
Live cells |
50 µM |
/ |
[98] |
ALP-33 |
0.05–1.05 U/mL |
/ |
0.012 U/mL |
/ |
/ |
377/572 |
0.0392 |
/ |
Colorimetric and fluorescent on-off-on |
Over expressed cancer cells & normal salivary gland cells |
25 µM |
/ |
[99] |
ALP-34 |
/ |
/ |
/ |
/ |
/ |
/ |
/ |
/ |
Turn-on fluorescence (NIR fluorescence) |
In vitro and live cells |
/ |
Osteoblast |
[100] |
ALP-35 |
0.01–2.5 U/mL |
/ |
0.0033 U/mL |
/ |
/ |
685/718 |
/ |
148.6 µM |
Turn-on fluorescence (NIR fluorescence) |
In vivo |
/ |
/ |
[101] |
4. Organo-Metallic-Based Fluorescent Probes
Seo et al. reported the fluorescein/hydrazine-based probe
ALP-33 (
Figure 30) to investigate ALP activity in cellular systems by measuring the consumption of trace amounts of PPi in the intracellular matrix
[99]. With this indirect strategy, the green emissive probe
ALP-33 was designed to detect Cu
2+/Ppi under physiological conditions in a sequential manner. In this sequential analysis,
on–off–on switching properties were observed upon sequential addition of Cu
2+ and PPi ions. In addition, dramatic color transitions occurred from colorless → yellow → colorless.
ALP-33 showed a characteristic absorption maximum at 370 nm in the UV–Vis absorption spectrum. However, upon addition of Cu
2+ ions, a new band at 446 nm was generated, making the solution an intense yellow color.
Figure 30. Plausible recognition mechanism of Cu2+/Ppi and intracellular ALP activity using ALP-33.
In a detailed investigation, endogenous ALP activity was monitored in normal salivary gland cells and cancer cell lines. By tracking the intracellular PPi, by employing a Cu
2+ entity, the alkaline phosphatase activity was monitored in the presence of a fixed dose of an inhibitor (Na
3VO
4) in ALP-overexpressing cancer cells and normal salivary gland cell lines. In the time and dose-dependent study, the scholars used specified amounts of
ALP-33–Cu
2+ complex for specified numbers of cells. Upon detailed analysis of PPi amounts produced in cancer cells at specified conditions, endogenous ALP activity was monitored at different time intervals. Intriguingly, complex-incubated cells (cancer and normal cells) showed bright green emission only in the presence of Na
3VO
4 (inhibitor). Relative signal intensities in cancer cells were higher than in normal cells due to rapid mitotic divisions, which resulted in the generation of large amounts of PPi in cells.
5. Nanomaterial-Based Fluorescent Probes
Lim et al. developed a new type of nanocomposite by formulating the doping of FDA-approved indocyanine green (IcyG), a fluorescent material, onto hydroxyapatite, followed by its surface modification through gold nanoparticles. This composite,
ALP-34 [100], was made for the recognition of ALP activity during the osteoblast differentiation process (
Figure 31).
Figure 31. Schematic representation of gold nanoparticles conjugated with fluorescent hydroxyapatite (ALP-34) for the detection of alkaline phosphatase activity.
Compared to organometallic complexes, nanomaterials are more promising candidates for the in vitro and in vivo recognition of ALP activity. This is because nano-sized functional materials usually show excellent cell transfusion capabilities, along with excellent photo-stability. Depending on surface functionalization, nanomaterials cellular localizations can be regulated. Even though not many fluorescent nano-materials have been reported for the in vitro, in cellulo recognition of phosphatase, and for their applications in cancer related systems, in near future, next generation nanomaterials could emerge as promising candidates for phosphatase-associated diagnostic tools for cancer and diagnostic tools for other diseases.
6. Miscellaneous
Ou-Yang et al. reported an NIR-based infinite coordination polymer nanoparticle (
ALP-35) (
Figure 32) for endogenous ALP activity detection in in cellulo and in vivo models
[101]. The rationally designed nanoprobe was constructed based on doping of fluorescent dye into a metal-ion-driven co-ordinational supramolecular nano-assembly. Accordingly, NIR emissive hemicyanine dyes were doped into Tb
3+-GMP, an infinite nano-co-ordinational polymer. Due to the proximity induced quenching in confined spaces in the metal nano-coordination polymer, NIR emissive behavior was diminished. However, upon dephosphorylation, self-assembled metal nanostructures gradually dissolved by releasing NIR dye. The nanoprobe showed a sharp absorption band at ca. 695 nm and weak emission centered at 738 nm upon excitation at 685 nm. Paradoxically, upon ALP-catalyzed dephosphorylation of GMP, there was bright NIR emission centered at 738 nm (~8-fold) and substantial hyperchromicity in the absorption spectrum.
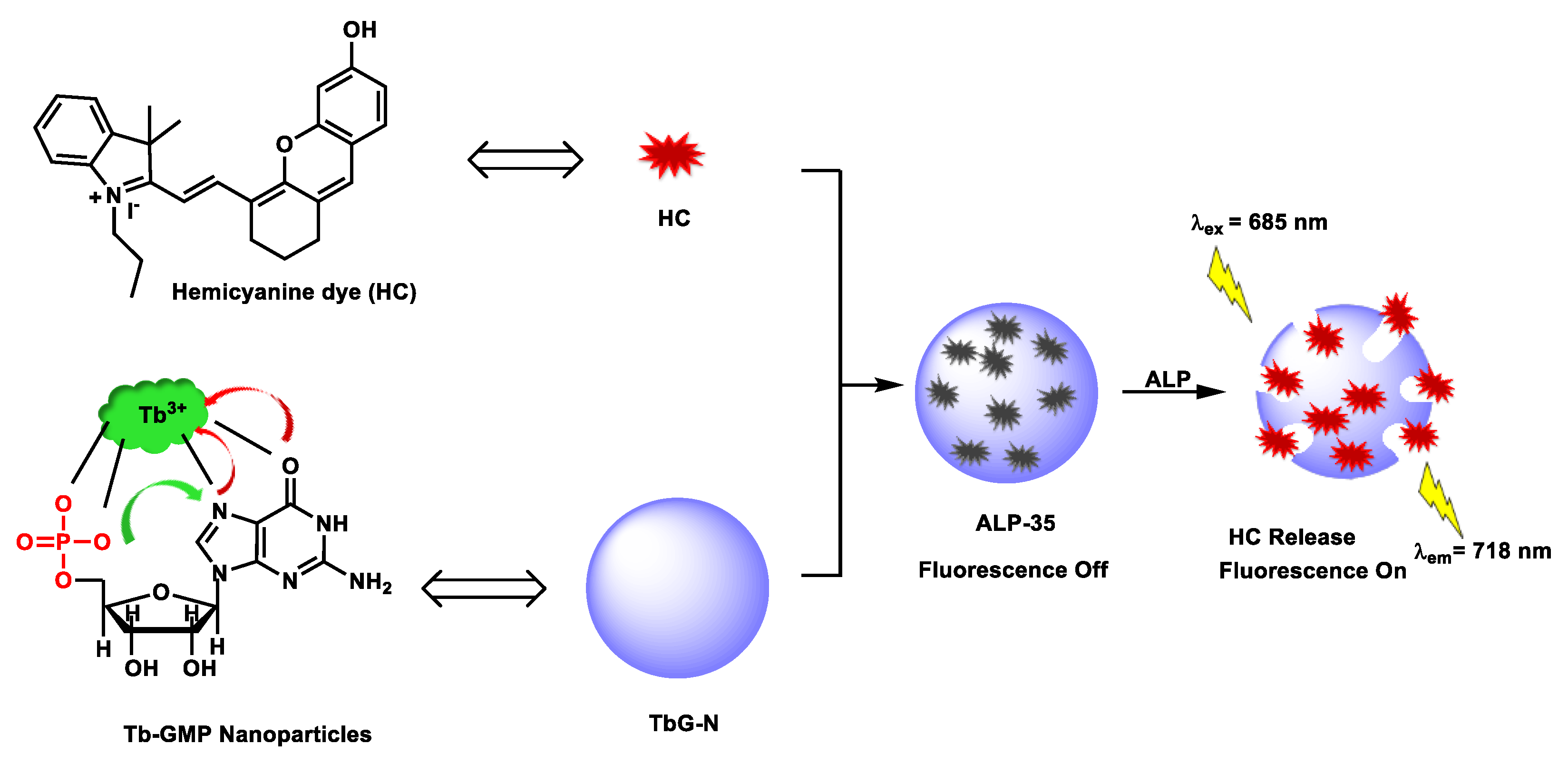
Figure 32. Schematic representation of the ALP-35 nanoprobe’s design and a plausible phosphatase recognition mechanism.
ALP-35 showed a good catalytic constant (kcat ~ 0.03 s−1) and the detection limit of 0.0033 U/mL towards phosphatase, and showed no interference from biologically relevant molecules, such as GOX, EXO and trypsin. Inhibitor assay studies with sodium orthovanadate (Na3VO4) showed an IC50 of 148.6 μM for ALP in similar experimental conditions.