The synthesis and applications of composites based on layered double hydroxides (LDHs) and nanocarbons have recently seen great development. On the one hand, LDHs are versatile 2D compounds that present a plethora of applications, from medicine to energy conversion, environmental remediation, and heterogeneous catalysis. On the other, nanocarbons present unique physical and chemical properties owing to their low-dimensional structure and sp2 hybridization of carbon atoms, which endows them with excellent charge carrier mobility, outstanding mechanical strength, and high thermal conductivity.
1. Introduction
Nanocomposites combining LDHs and carbon-based materials as building blocks have attracted a great deal of interest lately, particularly as electrocatalysts and photocatalysts. Several excellent reviews related to the synthesis, characterization, and application of LDH/carbon nanocomposites highlight their remarkable properties and performance for energy storage and conversion, environmental protection, and pollution abatement
[1][2][3][4][5][6][7]. Although less developed, the applications of LDH/carbon nanocomposites as nanofillers, non-enzymatic sensors, adsorbents for water remediation, and drug delivery systems have been also reported in several reviews
[8][9][10][11]. Some of them point out the use of LDH/carbon nanocomposites as catalysts in acid–base and redox reactions
[3][8][11][12]. However, there is now a remarkable number of publications reporting the high efficiency of LDH/carbon nanocomposites in these reactions.
This type of application was reported for the first time in 2005
[13][14]. MgAl-LDH/carbon nanofiber (CNF) composites were used in the base-catalyzed condensation of acetone, reaching specific activity four times higher than that of the unsupported MgAl-LDH. Moreover, impregnation of a MgAl-LDH/CNF composite with Pd led to a bifunctional catalyst achieving the single-stage synthesis of methylisobutylketone (MIBK) from acetone, with initial activity five times higher than the mechanical mixture of activated MgAl-LDH and Pd/CNF catalysts. Afterwards, various LDH/carbon nanocatalysts have been implemented, displaying highly successful performance compared to unsupported LDH-based catalysts for base-catalyzed C–C bond-forming reactions, as well as for oxidation and reduction reactions. Their higher efficiency than the single MgAl-LDH mainly results from the higher specific surface areas and porosity. Moreover, interactions between LDH nanosheets or metal nanoparticles (NPs) obtained from LDH precursors and nanocarbons improve electron transfer and avoid aggregation of the LDH or metal NPs. The LDH/carbon composites also exhibit high thermal and chemical stabilities. They generally retain the intrinsic properties of the individual LDH and carbon components with additional synergistic effects.
The hierarchical nanocomposites derived from a multitude of LDH compositions and 0D carbon dots (CD), 1D nanofibers (CF), single-walled carbon nanotubes (SWCNT) and multi-walled carbon nanotubes (MWCNT), and 2D graphene-like compounds give rise to a large family of materials.
The main characteristics of each type of carbon material have been extensively described
[15][16][17][18][19]. Graphene, particularly its oxidized or reduced forms, i.e., graphene oxide (GO) or reduced graphene oxide (rGO), is currently the most widely used nanocarbon component in LDH/carbon nanocatalysts. The use of nitrogen-doped graphene is also now emerging. The success of the graphene-like supports for the design of LDH-containing composites is partly based on their 2D structural compatibility, which allows intercalation. The resulting sandwich-type structures are rarely used as catalysts, where disordered arrangements with more accessible and dispersed active sites are desirable
[20][21]. GO and rGO are more likely chosen for their electronic and thermal conductivities, their ability to enhance the dispersion and avoid aggregation of the LDH, and to enhance the mechanical and chemical stability of the composites. CNF and CNT appear as the second type of nanocarbons used. More recently, carbon dots (CD) and nitrogen-doped carbon dots (NCD) have been considered promising to develop composites with improved basic properties, fast electron transfer through strong metal–CD interaction, and high mechanical resistance
[20][21][22].
2. LDHs and Carbon Materials
2.1. LDHs
LDHs are built up of brucite (Mg(OH)
2)-like layers, which consist of magnesium ions surrounded by six hydroxyl groups in an octahedral geometry where the divalent metal (M
2+) is isomorphically substituted by a trivalent one (M
3+). The excess of positive charge is balanced by intercalated anions coexisting with water molecules. The general formula of LDH can be written as [M
2+1−xM
3+x(OH)
2]
x+ (A
m−x/m)
x− · nH
2O, where M
2+ and M
3+ are di- and trivalent cations, respectively, and A
m− are the interlayer anions. The different compositions mostly found in the literature up to date are shown in
Figure 1. 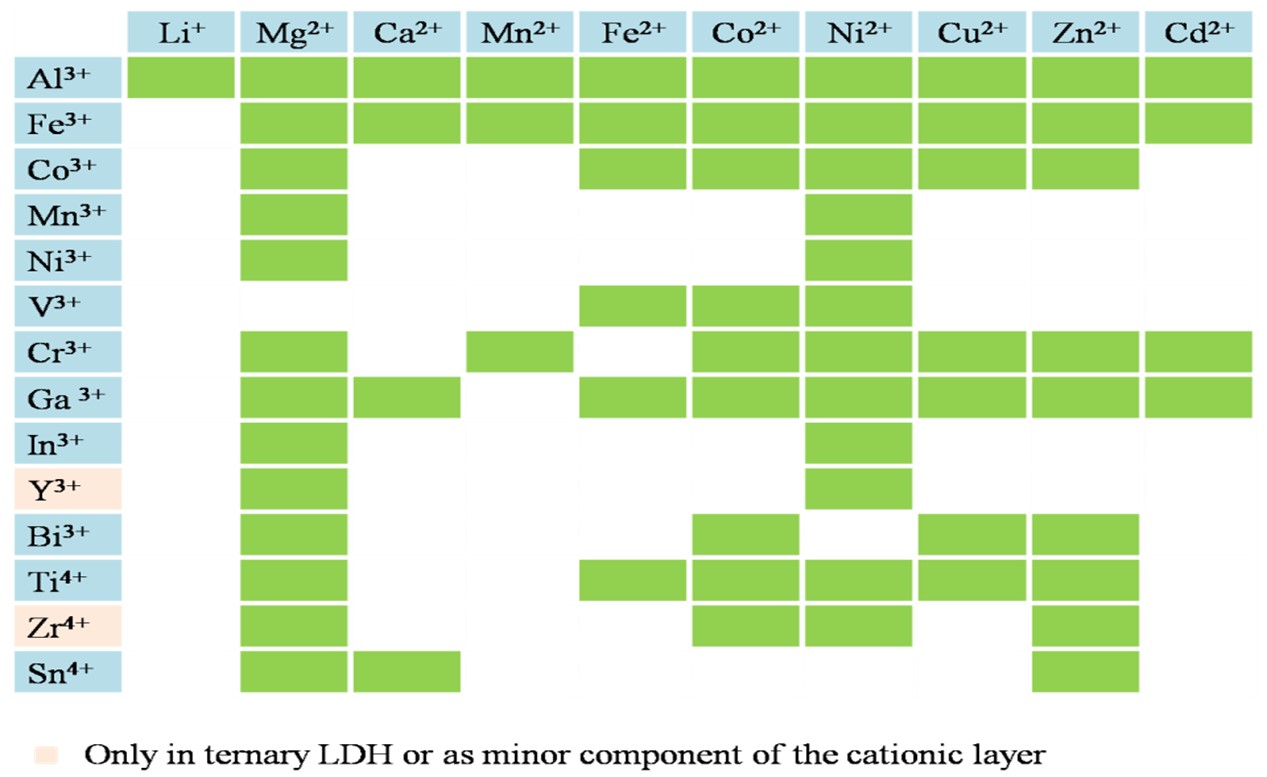
Figure 1. Possible cations combination in LDH.
The molar ratio M
3+/(M
2+ + M
3+) generally ranges from 0.2 to 0.33, although some different M
2+/M
3+ molar ratios have also been reported
[23]. LDHs exhibit remarkable versatility for the preparation of base- and metal-supported catalysts due to their variety of compositions and their activity either in the lamellar form or as layered double oxides (LDO) obtained by thermal decomposition. Moreover, they are highly suitable as precursors of metal-supported or multifunctional catalysts, with large specific surface areas and peculiar metal–support interactions
[23][24][25][26]. Different possible applications and synthesis of LDH are presented in
Table 1. However, LDHs are prone to particle aggregation, dissolution in liquid media, and sheet stacking, which causes low dispersion of metal NPs and hinders reactants’ accessibility to the active sites. This contributes to reducing the efficiency of the LDH-derived catalysts. Many of these drawbacks have been prevented by the dispersion of LDH-based catalysts on various supports. Among them, nanocarbons are increasingly used due to their complementary properties with LDHs.
Table 1. Synthesis methods and applications of LDH.
Synthesis of LDH
|
Applications of LDH
|
Common methods
Coprecipitation at low or high supersaturation
Urea hydrolysis method
Sol-gel method
Ion-exchange method
Rehydration/reconstruction
Miscellaneous methods
Salt-oxide method
Surface synthesis
Templated synthesis
|
Catalyst support: Ziegler-Natta, metal complexes, etc.
Catalysts: Hydrogenation, polymerization, polyalkoxilation, transesterification, condensation reactions, epoxidation, reduction, esterification, oxidation, oxidative dehydrogenation, water splitting, photocatalysis, etc.
Medicine: Antiacid, stabilizer, molecular container, etc.
Industry: Flame retardant, molecular sieve, ion exchanger, etc
Adsorbent: Anion scavenger, wastewater treatment, CO2 adsorption
|
2.2. Carbon Materials
Despite exhibiting the same general properties, i.e., electron conductivity, high mechanical strength, high thermal conductivity, and chemical inertness, each nanocarbon has specific properties. For catalytic applications, the nanocarbons should have a high surface area, suitable pore size, high graphitization degree, and strong interfacial coupling. In particular, 2D graphene-like materials, 1D carbon nanotubes (CNT), carbon fibers (CF) and nanofibers (CNF), and 0D carbon dots (CD) have been considered as components of the LDH/nanocarbons
[15]. These nanocarbon components are very versatile for surface modification and functionalization, which is necessary to ensure interfacial coupling with LDHs.
Graphene-like materials, i.e., graphene (G), graphene oxide (GO), and reduced graphene oxide (rGO), are the most reported materials involved in LDH/nanocarbon composites. G consists of a single layer of sp
2 and sp
3-hybridized carbon atoms organized in a 2D hexagonal lattice. The synthesis of graphene-like materials involves either bottom-up methods starting from carbon molecules or top-down methods using a carbon source, generally graphite. For catalytic applications, chemical synthesis is most suitable since it provides a reactive surface with high density of functional groups. Hummers’ method and its modified methods are the most common routes for graphite oxidation
[27]. The obtained GO can be easily exfoliated. Through a subsequent reduction step with a chemical agent or by ultrasonication or thermal or hydrothermal treatment, GO sheets are transformed into reduced graphene oxide (rGO).
A CNT can be considered as a rolled-up graphene sheet to form cylindrical molecules. They are classified as single-walled carbon nanotubes (SWCNT) and multi-walled carbon nanotubes (MWCNT) according to the number of rolled-up graphene layers forming the tubular nanostructure. CNTs present high strength, electrical and thermal conductivity and stability, and a high surface area, which makes them very attractive for catalytic applications.
CFs and CNFs exhibit structures and properties closely related and similar to those of CNTs. However, the geometry of CNFs, formed by regularly stacked truncated conical graphene layers, is different to that of CNTs
[19]. CNFs can be defined as linear filaments formed of sp
2 carbon atoms, giving a flexible, highly graphitic structure with lengths from several nanometers to microns. Similar to CNTs, CNFs present a high surface area and chemically active end planes, which facilitate its functionalization.
Carbon dots (CD), including carbon quantum dots CQD, graphene quantum dots (GQD) and N-doped carbon dots (NCD), and the CD/inorganic nanocomponents, are emerging as cutting-edge materials for the development of advanced catalysts. CD exhibit a size below 10 nm, whose structure is composed of sp
2 and sp
3 hybridized carbon atoms in the core and the outer part, respectively
[28]. A variety of top-down and bottom-up syntheses have been developed for producing CD with different characteristics.
The typical synthesis, structure, and properties of the nanocarbons used in the LDH/nanocarbon-derived catalysts are summarized in
Table 1. More extensive descriptions can be found in several recent reviews
[15][16][17][19][28][29][30][31].
Table 1. Properties and synthesis methods of different types of nanocarbons.