The emergence of SARS-CoV-2 variants with decreased susceptibility to the neutralizing antibody responses induced by currently available COVID-19 vaccines raises the possibility of breakthrough infections. Thus, alternative or complementary approaches are needed to develop vaccines able to induce a lasting immunological response. In the case of global public health emergencies, governmental vaccine design can benefit from a range of platform technologies, including conventional vaccines such as inactivated and live-attenuated vaccines, the innovative new class of DNA- and RNA-based vaccines and promising protein-based vaccines. Compared with conventional vaccines, molecular-based platforms may offer a more versatile tool against new emergent viruses, allowing fast, low-cost, and scalable vaccine manufacturing. Essentially, these platforms rely on the use of a system to deliver and present a new antigen (or a synthetic gene) to rapidly target an emergent pathogen. Currently, there are four different platforms used to develop viral vaccines: whole virus, nucleic acid-based, viral vectors, and protein and virus-like particles (VLPs). The choice of platform takes into account many factors, including the way the immune system responds to the specific viral infection, vaccination strategies and policies, and the best technology or approach to create the specific vaccine.
1. Whole Virus Vaccines
There are two types of whole virus vaccines: inactivated and live-attenuated viral vaccines. In inactivated viral vaccines, the virus is rendered noninfectious by using chemicals such as formaldehyde, radiation treatment, or heat
[1]; the viral genetic material does not replicate but is able to trigger the host immune response. In contrast, live-attenuated viral vaccines consist of weakened or attenuated viruses, which are able to replicate within cells and to activate innate immune responses
[1].
Despite both vaccines containing the whole or part of the virus, the quality of the immune responses they are able to trigger is different; indeed, inactivated vaccines can only provide a humoral immune response and need several doses over time in order to get protective immunity. In contrast, live-attenuated vaccines are more effective and induce a strong immune response, both cellular and humoral, with just one or two doses. However, in some cases, they can cause adverse events in people with weakened immune systems, in those with long-term health problems, or in people who received an organ transplant. In addition, they need to be kept cool, and therefore a significant logistical mode of distribution is needed in order to provide a vaccine with high efficacy and without logistical challenges during distribution
[2].
2. Nucleic Acid-Based Technologies: DNA and mRNA Vaccines
Nucleic acid-based technologies employ either plasmid DNA or mRNA coding for the antigen(s) of interest and are directly injected into the recipient, whether human or animal, with the antigen subsequently produced in situ by cells of the vaccinee
[3]. These vaccines are extremely versatile since they allow the direction of the in vivo assembly of encoded vaccine antigens, thus bypassing the requirement for in vitro antigen expression, assembly, and purification. In addition, the production of antigens inside the target cells allows the obtaining of post-translational modifications with a high degree of faithfulness
[3][4]. Despite DNA encoding, viral antigens suffer from low immunogenicity
[5], and nanotechnology-based platforms also provide innovative methods to improve specific delivery and sustainable release of antigens and to reduce off-target side effects
[6]. In particular, the introduction of adjuvants combined with immunoregulatory agents contributes to the control of improper immune stimulation and to the loss of bioactivity of immunostimulatory agents during circulation
[7][8], allowing the obtaining of DNA and mRNA vaccines capable of inducing both humoral and cellular immune responses.
2.1. DNA-Based Vaccines
DNA vaccines consist of plasmid DNA-encoding antigens that are expressed in host cells as native antigens with species-specific post-translational modifications
[9]. By using administration devices, such as a gene gun or electroporation, DNA vaccines must be introduced into the host cell nucleus; the vaccine DNA can then be transcribed into mRNA that in turn migrates into the cytoplasm, where it is translated into antigenic proteins. Antigen-presenting cells process these proteins and present them to lymphocytes that have become capable of killing pathogens and infected cells that express these target proteins.
Despite DNA vaccines being capable of eliciting both humoral and cellular immunity in theory
[7][8][10], the main challenge that limits clinical application is the blocking of their delivery via targeted immune cells which obstruct the stimulation of robust antigen-specific immune responses in humans
[7][8][10]. However, the immunological response can be enhanced by several techniques, including complexing the DNA with lipids or polymers in order to decrease the size for easy passage through the cell membrane
[7][8][10].
The use of DNA vaccines carries other disadvantages. The introduction of foreign genetic information into the nucleus of the transfected host cells is associated with future oncogenic potential, which is additionally enhanced by the long-term persistence of DNA plasmids upon injection
[7]. Another problem in vaccinated organisms can be the potential expression of specific antibiotic-resistance markers originating from antibiotic resistance genes used for the production of plasmid DNA, although these markers have been replaced in next-generation DNA vaccines. Finally, the expression of cytokines or the costimulatory molecules always used to enhance DNA immunogenicity might induce adverse effects, including inflammatory responses, autoimmunity, or immune suppression
[11]. Furthermore, despite advancements in adjuvant- and nanomaterial-based delivery systems, DNA vaccines fail to generate potent immunogenic responses
[7][8] and require repeated injections of large amounts of plasmid DNA
[7][10][12].
2.2. RNA-Based Vaccines
Unlike DNA vaccines, RNA vaccines are translated upon crossing the plasma or endosomal membranes, without the need for entering the nucleus. Two major types of mRNA vaccines were developed against infectious pathogens: conventional nonreplicating mRNA vaccines and self-amplifying vaccines (or viral replicons)
[3][13].
Nonreplicating (or nonamplifying) RNA vaccines are the simplest type and consist of small mRNA molecules encoding only the antigen of interest and containing 5′ and 3′ untranslated regions
[13][14]. Among the main advantages of using nonreplicating mRNA vaccines are the simplicity of the construct, the small molecular size, and the absence of any additional encoded proteins that could induce unintended immune responses
[13].
In contrast, self-replicating RNA vaccines are large mRNA molecules derived from specific viral genomes are commonly based on an
alphavirus or
flavivirus genome backbone that is defective in at least one structural protein gene but contains genes encoding the viral antigen as well as the viral replicase enzyme machinery that allows their self-amplification
[14].
Consequently, compared with conventional nonreplicating mRNA vaccines, self-amplifying RNA vaccines allow intracellular antigen-encoding RNA amplification and higher antigen expression levels
[14]. However, the bigger size of these vaccines makes manufacturing processes more difficult, with lower yields and an increased occurrence of abortive constructs. In order to act as a vaccine, exogenous mRNA has to enter the cytoplasm by crossing the plasma or endosomal lipid membrane, and once it is delivered into the cytoplasm of the host cell, it can be translated by the host translational machinery, producing the corresponding post-translationally modified antigen, thus mimicking an in vivo viral infection. Several studies showed that mRNA vaccines can efficiently trigger the activation of the innate immune system, also inducing strong adaptive immune responses
[15][16][17]. The intrinsic innate immune stimulation properties of mRNA can be further increased by implementing different mRNA formats and formulations. A more commonly used strategy to increase expression and immunogenicity is the delivery of mRNA in complex with additional components, such as the protamine-complexed mRNA format that has been shown to provide both strong antigen expression and efficient immunostimulation
[18][19][20].
The efficacy of mRNA vaccines can be further improved by adding safe complexing agents such as lipid- and polymer-based nanoparticles, which enhance uptake by cells, improve delivery to the translational machinery in the cytoplasm, and are also well tolerated
[21][22][23]. The most commonly used components for lipid-based nanoparticle formulations are represented by ionizable amino lipids, phospholipids, cholesterol, and lipid-anchored polyethylene glycol. Since mRNA vaccines are fully synthetic, they do not need a biological phase, thus being able to gain access to clinical trials in a very short time
[15].
However, despite naked mRNA being able to induce immune responses, RNA vaccines containing mRNA alone are not applicable for broad use as prophylactic vaccines since the ubiquitous presence of extracellular ribonucleases catalytically hydrolyzes RNA
[24]. Altogether, since the production process of mRNA is based on in vitro systems and does not require amplification in bacteria or cell cultures, the manufacturing of mRNA vaccines is a comparably shorter and simpler process to monitor. Furthermore, given that mRNA vaccines do not enter the nucleus, they avoid the potential risk of genomic integration posed by DNA-based vaccines. Most mRNA vaccines can be administered by different routes using conventional needle-based injections and, unlike DNA vaccines, without requiring any additional administration devices. Therefore, mRNA vaccines offer a flexible one-for-all, large-scale, rapid and cost-effective manufacturing process with fast turnaround times. These characteristics are very important to counteract pandemic threats that require a rapid response platform capable of producing protective vaccines in a short time frame in order to protect at-risk populations and to have an early impact on the progression of an outbreak.
3. Recombinant Viral Vector-Based Vaccines
Replicating recombinant vector vaccines consists of a fully competent viral vector backbone engineered to express an antigen from a foreign transgene
[24]. In the viral vectors, the synthetic gene encoding the antigen(s) is inserted into one of the specific viruses that usually have been engineered so that they cannot replicate in the human host. The virus is then grown in culture and used to deliver the synthetic gene during vaccination.
Recombinant viral vaccine vectors have been shown to induce robust and long-lasting immune responses, thus providing protective efficacy against a variety of infectious disease threats to humans and animals, including those with the zoonotic potential to cause global pandemics. Several types of human and animal viruses, including chimpanzee Ad, human Ad 5 and 26, the measles virus, vaccinia Ankara, the vesicular stomatitis virus, and the cytomegalovirus have been modified for use as vector-based vaccines
[25].
In particular, recombinant Ad vector-based vaccines have been shown to be very attractive as they induce both innate and adaptive immune responses in mammalian hosts.
Currently, Ad vector-based vaccines are being used to counteract numerous infectious diseases, ranging from malaria to HIV-1
[26]. Additionally, these vaccines can stably express inserts of up to 8 kb, supporting the expression of most target antigens, thus acting as multivalent or multipathogen vaccines
[27]. Furthermore, they support high viral yields at relatively low production costs. Ad vector-based vaccines are also able to induce potent antibody as well as T-cell responses, with variations in the immune response depending on the serotype employed
[28].
However, in some cases involving the use of human Ads as vectors, the widespread pre-existing immunity in the human population can inactivate the viral vector, thus inhibiting transgene expression
[29]. This issue has been met by developing Ad vectors of nonhuman origin or, as an alternative approach, by selecting rare serotypes with low prevalence in humans
[27][30].
Administration of viral vector-based vaccines can take place through different routes of administration, including intramuscular, intranasal, intradermal, and oral, depending of the target pathogen
[31][32][33]. Viral vectors induce infection-like stimuli within target cells, thereby enhancing potent immune responses without the need to add adjuvants
[34][35].
The valid strategies to achieve replication incompetency or attenuation in order to obtain a good safety profile, together with high yield production processes, support the use of these viral vectors for pandemic settings.
However, since viral vectors are genetically modified organisms, they represent a potential risk to human health and environment. Moreover, their use as attenuated vector-based vaccines could lead to high or persistent replication or to their integration into the host genome. In addition, since the production of these vaccines involves multiple steps and components, including cells and substrates, it requires extensive testing to exclude the presence of contaminants
[36]. These factors, together with the screening procedures to evaluate potential pre-existing immunity, make the production of viral vector-based vaccines a highly complex and cost-intensive process.
4. Recombinant Protein-Based Vaccines
Many recombinant proteins, realized through genetic engineering techniques, have historically been used as vaccine candidates
[37]. Protein subunit vaccines are made of one or more purified antigens from the viruses or bacteria of interest that are able to trigger the immune system and raise a protective immune response. The first subunit vaccine was approved in 1986 and directed against the hepatitis B virus (HBV) by including a recombinant viral surface antigen (HBsAg) able to self-assemble using
S. cerevisiae as an expression system. However, recombinant protein vaccines are, per se, poorly immunogenic and require association with a specific adjuvant(s) to increase the induction of long-lasting protective immune responses
[38]. Thanks to the absence of infectious components, these vaccines are considered safer compared with those derived from live viruses or recombinant genetic material
[39].
5. Virus-like Particles (VLPs)
VLPs are made of multimeric viral proteins which are similar to native viral particles but are not infectious and do not contain genetic material. Multimers of proteins are responsible for the formation of chimeric VLPs (cVLPs) that stimulate protective immune responses via activation of T and B cells
[40]. Because of poor immunogenicity, VLPs need to be complexed with adjuvant molecules. After being recognized as naïve molecules by the host immune system, they are capable of boosting immune responses. These types of vaccines have the advantage of being relatively safe, adaptable, and less expensive compared with other platforms
[41]. Depending on their structural composition, VLPs can be classified as nonenveloped (neVLPs) or enveloped (eVLPs) candidates. Both kinds can then be composed of native viral antigens only (homologous VLPs) or can contain a mixture of proteins from different sources to increase overall immunogenicity (heterologous VLPs)
[42].
6. Immune Effects of Viral Vaccine Platforms
Vaccination can contribute to preventing or controlling the spread of contagious viral diseases by activating the host immune system to induce long-term immune memory, a shown in Figure 1.
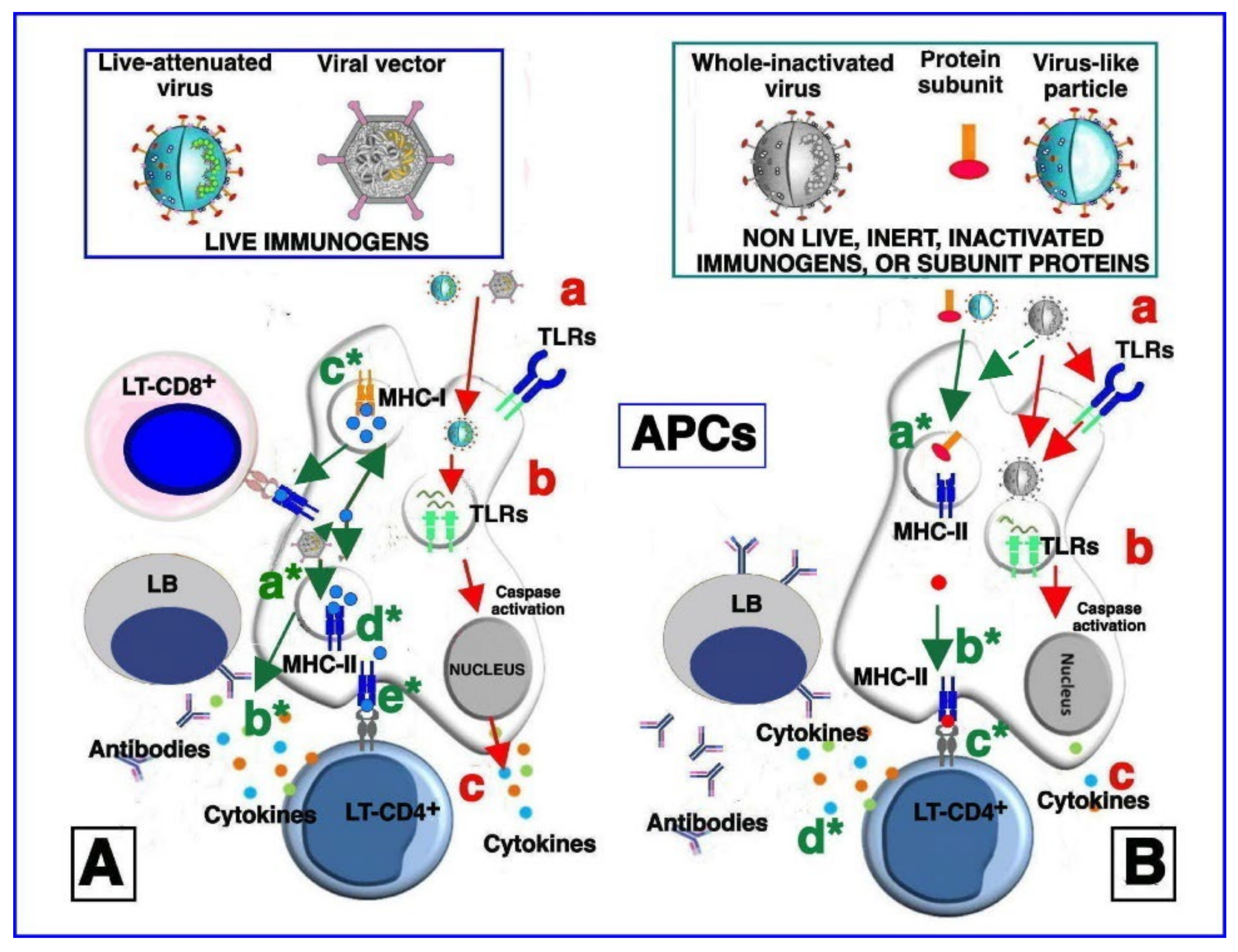
Figure 1. Simplified model of vaccine stimulation of the immune system. (A) Immune stimulation by live-attenuated viruses and/or vectored viral immunogens. After immunization, live-attenuated and vectored viral particles are endocytosed by antigen-presenting cells (APCs). Live-attenuated viruses can activate cell membrane pattern recognition receptors (PRRs) such as Toll-like receptors (TLRs) 2 and 6 (a). Upon entry, the live-attenuated viruses expose the nucleic acid and transcribe their genes, which, in turn, are sensed by endosomal TLRs (b). Activation of TLRs initiates signaling pathways culminating in the caspase pathway activation and production of pro-inflammatory and antiviral cytokines and chemokines (c). Immune stimulation by vectored viral immunogens may induce, via NOD-like receptor family pyrin domain-containing (NLRP) 3 pathway, inflammasome activation (a*) and cytokine production (b*). The transcribed vector-encoded transgene generates the immunogenic proteins (blue circles), which can then be proteosome-processed and associated with class I major histocompatibility complex (MHC-I) (c*) or with class II major histocompatibility complex (MHC-II) in endocytic vesicles (d*). MHC-I molecules loaded with transgenic epitopes translocate to the cell membrane, where they are recognized by antigen-specific CD8+ T cells (e*). Consequently, the infected cell is killed, and releases antigens in the extracellular space. In the same way, MHC-II molecules loaded with transgenic epitopes and translocated to the cell membrane are recognized by CD4+ helper T cells, which secrete cytokines and chemokines and further activate antigen-specific CD8+ T cells and B cells. Finally, stimulated B cells maturate into antibody-secreting plasma cells and/or memory B cells, as well as a portion of the stimulated T cells, which become memory cells (not shown). Overall, live immunogens are able to equally stimulate both humoral and cell-associated immune responses). (B) Immune stimulation by non-live (inert) inactivated vaccines, protein subunits, and virus-like particles. Immunization with antigens, inoculated together with the adjuvants that are added to vaccine formulations induces cytokine production from local cells. Cytokines in turn activate and/or attract APCs to the immunization site. In addition, the antigens may directly activate APCs through binding to cell membrane TLRs (a). The inactivated viruses are phagocytized by APCs and nucleic-acid traces inside the phagosomes and may activate endosomal TLRs (b), leading to the production of cytokines and chemokines (in a smaller amount, compared to (A) (c). The antigens contained in the inert vaccines in protein subunit formulations and in virus-like particles, after the entry in the call, are also degraded inside the endocytic vesicles, loaded onto MHC-II molecules (a*), and presented to CD4+ T cells (b*). Activation of CD4+ T lymphocytes leads to production of cytokines and chemokines, which induce the activation of antigen-specific B cells (c*), which maturate into antibody-secreting plasma cells (d*) and/or memory B cells. In general, inert antigens, such as nonlive inactivated vaccines, protein subunits, and virus-like particles, induce potent humoral responses and low-to-moderate T-cell responses. Activation of CD8+ T cells by inert antigens occurs through alternative pathways that are not depicted in this figure. The stimulation processes described and depicted in steps (a), (b), and (c) is reported less frequently and is less potent than stimulation by live immunogens (A), as depicted in (B) panel).