Ischemic stroke is a leading cause of death and disability worldwide. Following an ischemic insult, cells undergo endoplasmic reticulum (ER) stress, which increases the ER’s protein-folding and degradative capacities and blocks the global synthesis of proteins by phosphorylating the eukaryotic translation initiation factor 2-alpha (eIF2α). Phosphorylation of eIF2α is directly related to the dynamics of stress granules (SGs), which are membraneless organelles composed of RNA-binding proteins and mRNA. SGs play a critical role in mRNA metabolism and translational control. Other translation factors are also linked to cellular pathways, including SG dynamics following a stroke. Because the formation of SGs is closely connected to mRNA translation, it is interesting to explore the relationship between SG dynamics and cellular outcome in cases of ischemic damage.
1. Introduction
Ischemic stroke remains one of the leading causes of mortality and disability in Europe
[1]. Following an ischemic insult, two large areas can be distinguished: the ischemic core, infarcted tissue with irreparable damage; and the penumbra area, surrounding the ischemic core, which contains hypoperfused tissue that is still viable
[2]. Therefore, avoiding the transformation of penumbral into infarcted tissue is a key step in overcoming neuronal damage, as well as improving outcomes in patients. Following the occlusion of a blood vessel, the pathological paths of the ischemic cascade are activated, which eventually leads to neuronal death by necrosis or apoptosis
[3]. A hypoxic environment triggers glutamate excitotoxicity through a massive release of glutamate after injury, which causes neurotoxicity by binding to N-methyl-D-aspartate receptors (NMDAR), thereby promoting the entry of large amounts of Ca
2+ into neurons
[3]. High concentrations of intracellular Ca
2+ produce neuronal damage by increasing endoplasmic reticulum (ER) stress and the number of reactive oxygen species (ROS), and by causing a depletion of ATP-dependent processes
[3].
The translation of protein-coding nuclear genes is a vital cytoplasmic process necessary for development and cellular homeostasis
[4][5] (
Figure 1).
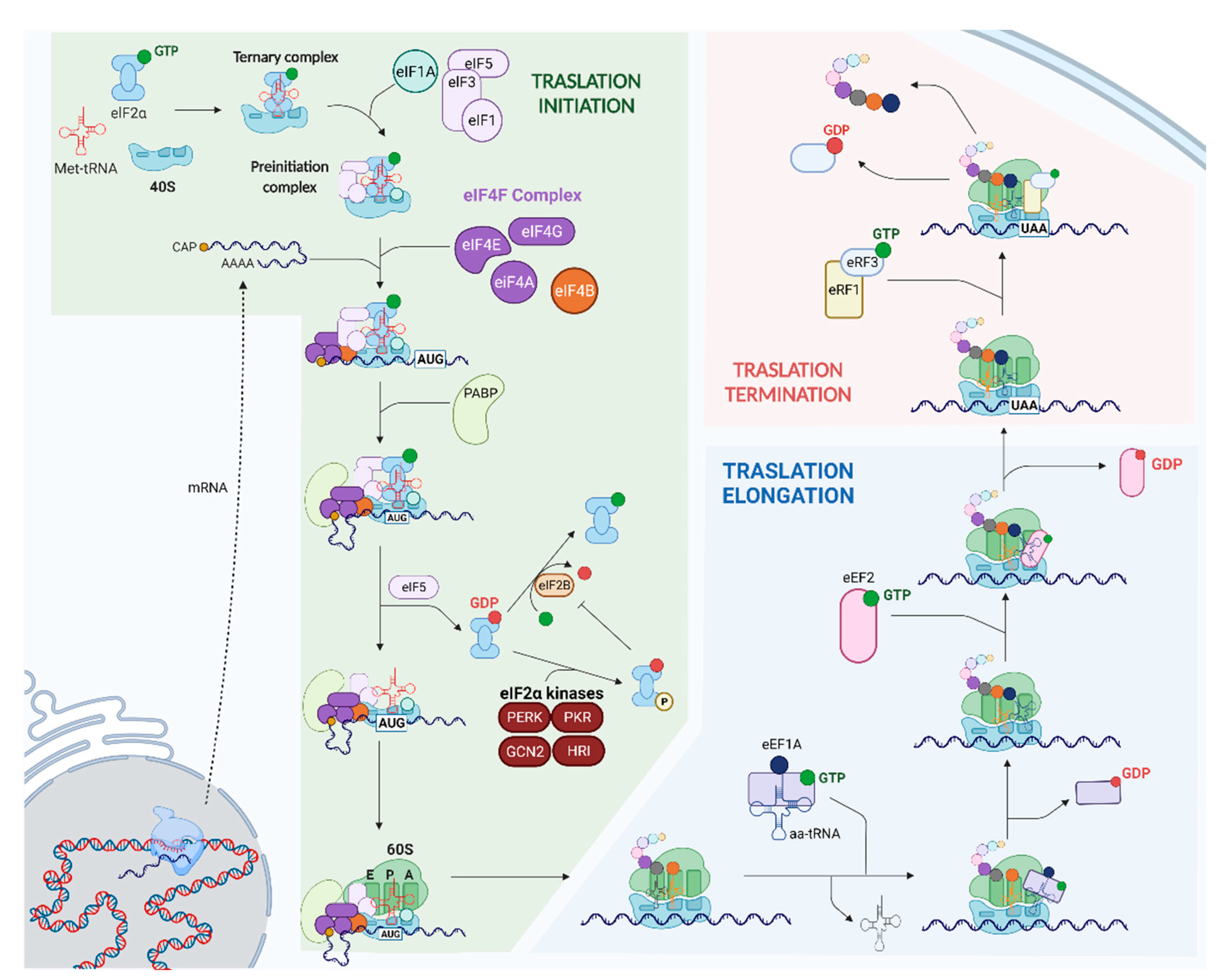
Figure 1. Assembly of the preinitiation complex (PIC), eIF4F complex (eIF4E, eIF4G, and eIF4A), and eIF4B in the 5′ cap of the mRNA triggers initiation (dashed arrow). Once a start codon (AUG) is found, eIF2-GDP is released, the 60S ribosomal subunit joins the PIC, and an elongation-competent 80S ribosome is formed. eIF2-GDP can undergo incapacitating phosphorylation that blocks protein synthesis under stress conditions. During elongation, the ternary complexes of eEF1A-GTP-aminoacyl-tRNA bind to the ribosome at the A site, increasing the length of the aminoacidic chain. Several rounds of elongation occur until a stop codon is reached, at which point the complex eRF1-eRF3-GTP recognizes it and translation is terminated.
It is well-established that ER stress plays an important role in the pathophysiology and outcome of ischemic stroke
[6]. ER stress induced by ischemic injury triggers the phosphorylation of eIF2α to block the global synthesis of proteins and, subsequently, to increase the protein-folding and degradative capacities of the ER
[7][8]. So far, four kinases have been described as able to phosphorylate eIF2α, with a major role played by EIF2AK2 (PKR) and EIF2AK3 (PERK) in the ischemic event
[8][9] (
Figure 2).
Figure 2. The phosphorylation of eIF2α and disassembly of the eIF4F complex are the main pathways blocking protein translation in cases of endoplasmic reticulum (ER) stress. The kinases PERK, PKR, GCN2, and HRI can phosphorylate and, therefore, inhibit eIF2α. In both cases, RNA-binding proteins (RBPs) such as TIA1 or G3BP1, among others, interact with the incomplete PIC and promote SG formation (dashed arrows). Importantly, the nucleated SGs mature by adding other RBPs that attach to existing mRNAs and/or bring new mRNAs.
Healthy neurons express many components of SGs, which can be visibly detectable as granules or present in subdetectable forms. Emerging findings show that SGs can assemble in both the cell bodies and axonal compartments and exhibit dynamic changes in response to damage
[10]. SGs may have evolved in order for cells to respond adaptively to transient challenges, but under chronic conditions, persistent SGs are considered a potential trigger for cell death.
2. SG Dynamics following Cerebral Ischemia
2.1. Changes in RBP Expression
Recently, proteomics and immunohistochemical studies have shed light on the dynamics of several actors that mediate SG assembly after cerebral ischemia
[11][12]. Cortical protein aggregations of RBPs, such as heterogeneous nuclear ribonucleoproteins A0 and A1 (hnRNPA0 and hnRNPA1, respectively), heterogeneous nuclear ribonucleoprotein P2 (FUS), and TAR DNA-binding protein 43 (TDP-43), were found in a mouse model of cerebral ischemia induced by transient middle cerebral artery occlusion (tMCAO)
[11]. Importantly, these aggregations of hnRNPA0, hnRNPA1, FUS, and TDP-43 were present at 1 h after reperfusion, but dissolved after 24 h
[11]. All RBP aggregations were irreversible when a permanent MCAO model was used
[11]. This seems to indicate that reperfusion is needed to dissolve RBP aggregations
[11]. Increased levels of nuclear and cytoplasmic TDP-43 were found, with a peak at 48 h post-injury, in the neurons and glia of rats with subarachnoid hemorrhages
[13].
An abnormal cytoplasmic accumulation of RBPs may underlie cellular pathways related to neurodegeneration. Thammisetty et al. demonstrated that the aging-mediated accumulation of cytoplasmic TDP-43 leads to modifications that make it a dysfunctional protein
[14]. The higher cytoplasmic immunoreactivity of truncated TDP-43 was correlated with a larger ischemic lesion, increased neuronal death, and enhanced inflammation at 72 h post-reperfusion in older mice compared with younger ones, both groups having tMCAOs
[14]. In addition, another research using a rat model of transient focal cerebral ischemia found that damaged neurons displayed cytoplasmic translocation of TDP-43
[15]. TDP-43 was mainly colocalized with ubiquitin granules, showing higher levels of apoptosis after 24 h of reperfusion
[15]. Overall, the inability of TDP-43 to return to the nucleus triggers the creation of pathogenic forms of TDP-43 that partially underlie a worse outcome following ischemia, even when blood supply is restored. As for other chronic neurodegenerative diseases
[16], aging appears to have a key role in the outcome following ischemia by further increasing SG-mediated neuronal death.
To summarize, RBPs are moved from the nucleus to the cytoplasm right after the onset of cerebral ischemia; there, they trigger the formation of SGs and protect mRNAs from ischemic events. Reperfusion seems to be a key step for RBPs to return to the nucleus, which allows for the restoration of protein synthesis once the ischemia is resolved. A lack of restoration of blood flux causes severe neuronal damage by promoting prolonged translation arrest. Considering their implications in the relevant pathophysiologies, both processes are good candidates with which to find either biomarkers or therapeutic targets following ischemic stroke.
2.2. Modulation of RBP Function and Expression
The RNA-binding protein motif 3 (RBM3) is a well-known cold shock protein with beneficial roles following a stroke in both animal models and humans
[17][18]. Recently, the interaction between RBM3 and G3BP1 was suggested to mediate the survival of both the PC12 cell line and rat primary cortical neuronal cultures in an in vitro model of ischemia
[19]. In particular, RBM3–G3BP1 union might increase the ratio of SGs by facilitating their nucleation and formation, leading to a reduction in the rate of cell death
[19]. Another RBP related to SGs, called argonaute RISC catalytic component 2 (AGO2), was found to colocalize with G3BP1
[20]. AGO2, a protein mainly involved in microRNA (miRNA) maturation, was described as interacting with G3BP1 in the cytoplasm of microglial cells undergoing in vitro ischemia
[20].
2.3. Endothelial Cells and SG Dynamics
Endothelial cells from mice with tMCAOs displayed increased levels of TDP-43, mostly located in the cytoplasm at 24 h following the onset of ischemia and returning to the nucleus at 48 h
[21]. In addition, in vitro experiments revealed that the accumulation of TDP-43 in the cytoplasm of endothelial cells led to a reduction in the number of tight junctions and the inhibition of their migration capacity (negatively affecting BBB integrity and permeability); although it also increased their proliferation (promoting BBB repair)
[21]. Given that TDP-43 is translocated earlier to the cytoplasm and is involved in SG formation
[22], it can be hypothesized that TDP-43 acts in a similar way in endothelial cells following ischemia. Although the first research presented both harmful and beneficial outcomes, more studies are needed in order to better elucidate the mechanism/s underlying SG formation and vascular outcome after stroke. No publications were found regarding the presence of SGs in pericytes after stroke. As previously mentioned, this is a new field to investigate, as information on SGs, strokes, and the BBB is extremely scarce or non-existent.
3. Two Different Ways SG Dynamics Act in Triggering Neuroprotection after Ischemia
In the 1980s, it was observed that, under ischemic conditions, neurons from the hippocampal CA1 region undergo an irreversible inhibition of translation, which results in neuronal death; this is in contrast to resilient CA3 neurons
[23][24][25]. Several studies have highlighted the relationship between SGs and the different survival outcomes seen in CA1 and CA3 neurons
[26][27][28]. Following 10 min of cardiac arrest and a subsequent 10 min of reperfusion, there was an increase in SG assembly (assessed by cytoplasmic colabeling of TIA1 and S6, a marker of the small ribosomal subunit 40S) in rat CA3 neurons, but not in the CA1 region
[28]. This value for CA3 neurons returned to normal conditions after 90 min of reperfusion, whereas CA1 neurons showed a later colabeling of TIA1 and S6 at 4 h post-reperfusion
[28]. This SG assembly was not triggered by eIF2α phosphorylation, but by a negative altering of the eIF4F complex
[26]. Given that SGs are sites of inhibited translation, it is feasible that the later SG assembly seen in CA1 neurons underlies their irreversible inhibition of translation
[28]. An in vitro research, using the hippocampal neuronal cell line HT22, reported that the inability of TIA1 to interact with its mRNA targets provokes apoptosis under oxidative stress
[29]. Accordingly, the oxidation of TIA1 may interfere with its protective role of binding mRNAs and promoting temporary SG assembly through a reduction in housekeeping protein synthesis under stress conditions
[29]. Another in vivo research showed a hypothetical mechanism connecting SGs and the delayed death of CA1 neurons
[27]. The immunohistochemical coexpression of SGs (TIA1 + S6 co-labeling) and ubiquitin-labeled protein aggregates was found in the CA1, but not the CA3, neurons of rats undergoing bilateral carotid artery occlusion after 2 days of reperfusion
[27]. Therefore, it was suggested that these protein aggregates impair SG dynamics by impeding their disassembly
[27]. This event could be related to the delayed translation of the heat shock chaperone 70 (Hsp70), detected in CA1 neurons but not CA3 neurons
[27][30]. Notably, the preischemia application of cycloheximide (a drug that prevents SG assembly) was able to increase the survival of CA1 neurons after 3 days of reperfusion
[26].
Overall, it seems that the dysregulation of the equilibrium between SGs and translating polysomes partially underlies the delayed death of CA1 neurons. It hypothesizes that the ischemia-mediated inability of TIA1 and/or delay in the formation of TIA1-assembled SGs may impede the protection of translating mRNAs under stress conditions in CA1 neurons. These events, along with the interaction of SGs with ubiquitinated protein aggregates, lead to irreversible arrested translation and, eventually, neuronal death. In contrast, the cycloheximide-induced trapping of mRNAs onto polysomes during ischemia inhibits SG assembly and their subsequent binding to protein aggregates, which results in higher CA1 neuron survival. However, the ‘kidnapping’ of some mRNAs such as HIF-1α is also necessary to promote neuroprotection under ischemic conditions. Therefore, these studies highlight the necessity of assessing the different roles of other RBPs in the formation of SGs, as well as their relationship to ischemia-mediated cellular pathways, as they are clearly involved in neuronal fate following damage.