1. Introduction
Wood–plastic composites (WPCs) entered the market in the 1990s; they are usually composed of up to 50%
w/
w of wood flour, thermoplastic polymers (polypropylene, polyethylene, polyvinyl chloride etc.), and small amounts of additives such as dyes or coupling agents
[1]. WPCs are most often used in construction and flooring, replacing lumber, wood-based boards (WBBs), ceramic tiles, or metals. WPCs can be designed for specific performance requirements, taking into account the advantages of the natural strength of the wood and the hydrophobic characteristics of the plastic component. The possibility of use of wood waste fillers as well as waste and recycled plastics reduces the environmental impact of the material, and also improves the circular economy
[2][3][4]. Other advantages of WPCs are their ease of maintenance, improved durability, and substantial service lifetime
[5]. The large weight share of polymers enables the shaping of WPC elements by moulding. Moulding allows the formation of complex shapes that would be very costly or even impossible to produce with pure lumber, wood-based boards (WBBs), or stone/ceramics. Furthermore, ready-made WPCs may be further shaped using the same tools as conventional lumber.
However, WPCs are also not free from disadvantages. The large weight share of the polymer matrix also predetermines the mechanical properties of the WPCs, which are close to those of their base polymers; therefore, they are poorer than (for example) raw wood. Another negative property that has been observed in WPCs is their susceptibility to environmental degradation. This type of degradation can occur as a result of various factors, including temperature, air quality, moisture (the polymer matrix does not fully exclude water interaction), microorganisms, light, high-energy radiation, chemical agents, and mechanical stress
[6][7]. These factors can decrease the aesthetic quality (discoloration) and mechanical strength of WPCs
[1][8][9]. Moreover, evidence has been seen of the presence of fungal attack on WPC boards that are exposed to natural weathering
[10][11].
Even taking account of these issues, the current wide use of WPCs, coupled with their composite nature, favours the development of research in this area. Very often, the studies are extended to the use of other cellulose-rich materials (e.g., milled straw, plant shells, or bamboo) as fillers
[12]. The latter composites are referred to as natural fibre–polymer composites (sometimes WPCs are classified as subgroup of NFPCs). NFPCs are not yet widely applied, as up to this point their performance has often been found to be less than satisfactory
[12]. However, new studies in this area show very encouraging results
[13][14][15][16][17][18]. Other studies focus mainly on the improvement of the performance of the WPCs, utilisation of new types of matrices, new manufacturing techniques, and an increase in the range of applications
[19][20][21][22][23]. One of the notable interesting areas of study is in the enrichment of the WPC and NFPC composites with carbon nanomaterials, such as carbon nanotubes (CNTs) and graphene.
Both carbon nanotubes and graphene are nano-allotropes of pure carbon
[24][25]. The structure of both is based on a planar hexagonal lattice of sp
2-bonded carbon atoms (
Figure 1a), i.e., every carbon atom forms a strong covalent bond with three neighbouring carbon atoms, and a much weaker pi bond is formed by the atoms laterally by their fourth available electrons distributed in
pz orbitals perpendicular to the hexagonal plane. This atomic structure imparts both CNTs and graphene with extremely high mechanical strength and stiffness, as well as superior electrical and thermal conductivity
[26]. Additionally, both of these nanomaterials are lightweight
[27], superhydrophobic
[28][29], chemically resistant
[30], and environmentally friendly, as they may be synthesised from greenhouse gases such as methane or CO
2 [31][32].
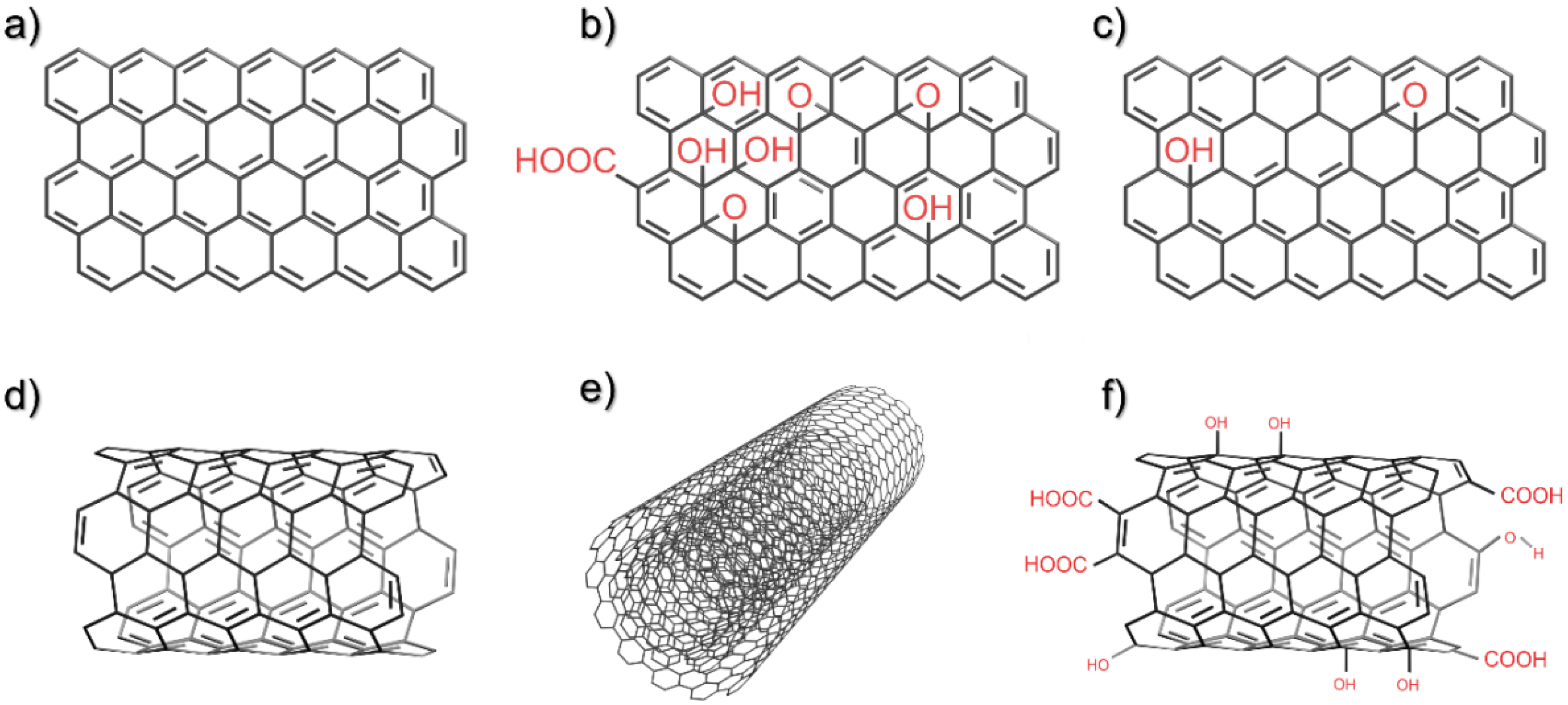
Figure 1. Structure of (a) graphene, (b) graphene oxide, (c) reduced graphene oxide, (d) single-walled carbon nanotube (e) multiwalled carbon nanotube, and (f) functionalised carbon nanotube. Annotation indicates where the structure is not pure carbon.
Graphene is a 2D structure that, in theory, is one atomic layer thick (
Figure 1a). In practice, the name graphene often refers to the whole family of two-dimensional carbon nanomaterials. The material to mention first in the context of WPCs is graphene nanoplatelets (GNPs); these are multilayered pure graphene structures often produced by the exfoliation of graphite, or via the chemical vapour deposition (CVD) process. Although the properties of GNPs may not be superior to those of single-layer graphene, they are sufficient to improve the properties of many polymer composites
[33]. GNPs are also quite inexpensive, easy to handle, and are produced and sold in large quantities. A further material is graphene oxide (GO) (
Figure 1b), which is a disordered single- or multilayered graphene functionalised with epoxy bridges, hydroxyl groups, and carboxyl groups
[34]. GO is electrically insulating, and has a much poorer mechanical performance than graphene/GNPs, but it is also a chemically active and hydrophilic structure; this enables dispersion in water and a uniform distribution in many polymers
[35]. Finally, reduced graphene oxide (rGO) is a material formed by the chemical reduction of GO. This process partially restores the electrical and mechanical properties of the graphene via the removal of most of the functional groups and the recovery of the sp
2 carbon–carbon bonds (
Figure 1c)
[36]. Often, the reduction process is performed after the composite manufacturing process, so as to benefit from the chemical reactivity of GO during manufacture and the electrical and mechanical properties of graphene in the final product.
Carbon nanotubes are one-dimensional structures of seamlessly rolled-up graphene sheets; they may be referred to as single-walled carbon nanotubes (SWCNTs) if made of a single layer of graphene (
Figure 1d), or multiwalled carbon nanotubes (MWCNTs) if two or more SWCNTs are encapsulated within one another (
Figure 1e). SWCNTs are often characterised as having better properties than MWCNTs, but the MWCNTs can be produced inexpensively and on a large scale
[31]. The CNTs may also be produced in a functionalised form to facilitate the dispersion of these structures in polymer matrices (
Figure 1f)
[37]. As 1D structures, the CNTs have nanosized diameters, and are most often nano- or micrometre lengths. In composites, CNTs form percolation networks more easily than graphene, but in some processes it is more difficult to obtain their high loading fractions
[38][39].
Both graphene and CNTs have been widely used in composites
[40]; they have been tested as fillers in many types of matrices, including polymers
[39][41], metals
[42], and ceramics
[43]. Polymer composites were actually the first major area in which CNTs and graphene found commercial applications. Both nanomaterials have been used to enhance mechanical properties
[44], improve thermal stability
[45], decrease flammability
[46][47], introduce electrical and thermal conductivity
[39], and much more
[48]. The applications of these composites include strong and lightweight construction materials
[43], electromagnetic shielding
[49], antistatic surfaces
[50], sensors
[51], components such as supercapacitors, etc.
[52]. It may therefore be expected that a combination of WPCs and other NFPCs with CNTs and/or graphene may produce materials with very interesting performance parameters, suitable for completely new applications.
2. Applications
As well as improving the performance of WPCs in current applications, the enhancement of WPCs with new functionalities can also be considered, allowing them to be used in completely new areas. For example, as indicated by Rajan et al.
[53], an increase in the electrical conductivity may render the WPC’s surface antistatic and simply prevent static electricity buildup in WPC constructions, or it may enable the use of WPCs in electronic packaging. Another application in this area may be the electromagnetic shielding studied by Chen et al.
[54].
Moreover, WPCs filled with CNMs could potentially be used as sensors for temperature, water, or bending
[55], although to the best of human's knowledge they have not been tested for these applications to date.
Another property that may be utilised in new applications is thermal conductivity. Lu et al.
[56] studied the thermal properties of wood–polymer composites enriched with GO, with a view to creating a solar thermal energy storage device. The authors designed a shape-stabilised phase change material (SSPCM) composed of polyethylene glycol (PEG)-based polyethylene glycol–polyurethane (PU), wood fibres, and graphene oxide. The PEG-based PU was used as a phase change material with a high energy storage density, with the wood fibres acting as a scaffold, while the GO enhancement increased the thermal conductivity and light absorption. As a result, a device with good thermal stability and a light–thermal conversion performance was produced.
3. Practical Considerations, Key Challenges, and Future Work
Currently, WPCs are widely applied, and are mostly used in indoor and outdoor furniture and flooring, frames, fences, etc. The general improvement in their properties, such as increasing their strength, or decreasing their water uptake or flammability, may definitely be of interest to the current industry. Naturally, the obstacles here may be the introduction of CNMs into industrial production and the price of the final product. However, an analysis of market reports for carbon nanotubes shows that the polymer-CNT composites currently constitute the largest share of the market
[57]. This indicates that the polymer industry is already well acquainted with carbon nanomaterials and the developed procedures for their incorporation into polymers, and these could be transferred to the industrial WPC production lines.
The initial estimations of pricing indicate that, assuming the current market prices of CNTs, GNP, and GO, as presented in Table 1, for low weight percentages of CNMs the price of a 1 m2, 2 cm thick WPC would not be increased significantly. Conversely, for high weight percentages (10 wt.%), the current costs are estimated to be outside of commercial viability. However, taking into account that most studies use low filler contents, and that 1 m2 of WPC costs USD ~100, it can already be found that the use of CNTs and graphene is quite feasible.
Table 1. Estimated prices of CNM additives to WPC.
CNM |
Manufacturer |
Price per 1 kg (USD) |
Estimated Price of CNM per 1 m2 of 2 cm Thick WPC (USD) |
with 0.1 wt.% CNM |
with 10 wt.% CNM |
CNTs |
Nanocyl SA |
120 |
1.44 |
144 |
GNP |
Cheap Tubes Inc. |
500 |
6.00 |
600 |
GO |
Graphenea |
3300 |
39.60 |
3960 |
However, before CNM-enriched WPCs or NFPCs can enter the market, there are still some significant scientific challenges that need to be addressed.
Going forward, in future studies, a much more pragmatic approach to experimental planning needs to be adopted. It would be useful to consider the vast corpus of knowledge gathered in the manufacture of pure CNM and polymer composites
[58][59][60][61], and to combine this with the state-of-the-art expertise already existing in the production of high-performance WPCs and NFPCs
[62][63][64]. Such analysis could potentially help with the choice of specific CNMs, wood particles, compatible polymers, and methods of manufacture, as well as avoiding such issues as agglomeration or poor adhesion of components. The performance of modelling studies could also provide valuable insight.
It would also be useful to look at aspects such as density, which is a very basic property of every composite. To date, only Kumar et al.
[57] and Rajan et al.
[53] have provided data showing density changes with the content of CNMs. Kumar et al. showed that the density decreases linearly with an increase in GPC content, while Rajan et al. found the opposite trend. These discrepancies may be related to many factors, including large variations in the density of the chosen CNMs and issues related to the manufacturing of the composites, e.g., the formation of voids in the composites. Analysis of the density data could therefore be useful in the analysis of the quality of the composites, as well as in understanding the mechanisms responsible for their formation.
Taking into account the practical applications of WPCs and NFPCs, it would be also necessary to perform more studies on the weathering of hybrid samples. Apart from the UV exposure tested by Peng et al.
[65] and the full water immersion effects studied by several authors (
Section 2.4), weather-related degradation agents may include heat, humidity, rain erosion, freezing, and thawing. All of these factors separately or acting collectively may impact the mechanical, physical, and chemical properties of hybrid composites and, therefore, may change their visual appearance (e.g., colour, gloss) and their surface topography (e.g., roughness, surface microstructure), as well as affecting their strength, stiffness, dimensional stability, and overall service lifetime. Other degradation factors that should be considered, and have not been taken into account thus far, include fungi and mould, as well as decay/staining by air pollutants and saltwater.
More studies should also look into the issue of biodegradation. The only study dealing with this aspect to date, by Yaghoobi et al.
[66], showed that enriching NFPCs with MWCNTs decreases the rate of biodegradation. This could be a very beneficial phenomenon; however, it could also be considered as a drawback when targeting the production of biodegradable composites
[67][68].
Finally, it may be interesting and helpful to test the hybrid composites for acoustic properties, coatability, oil staining susceptibility, wear patterns, sand erosion, and performance under drilling. All of these properties are dependent on the specific application of the designed composites.
4. Conclusions
In summary, to date, a large variety of studies on WPCs/NFPCs hybridised with carbon nanomaterials have been presented. The composites were prepared using different types and amounts of wood flour and other forms of natural fillers, such as oil palm shell powder, kenaf fibres, bamboo mats, etc. The matrices were based on both thermoplastic and thermoset polymers. The composites were prepared both with and without additives such as MAPP and MAPE compatibilizers or selected hardeners. Various types of manufacturing techniques were also used, including twin-screw extrusion, compression moulding, and laser sintering. The WPCs/NFPCs were hybridised with graphene nanoplatelets, graphene oxides, MWCNTs, and functionalised CNTs, as well as CNM masterbatches.
Inclusion of the CNMs in the WPCs and NFPCs enabled the introduction of a relative improvement in the mechanical properties of the studied composites, such as tensile strength, flexural strength, and impact strength. The hybridisation with CNMs also enabled an increase in thermal conductivity. Furthermore, the addition of CNMs rendered WPCs electrically conductive, improved their thermal stability and decreased their flammability, increased their photostability, decreased their water absorption, and improved their foaming efficiency. However, the analysis of the researches showed that the level of improvement differed significantly between the hybrid WPCs/NFPCs, and that in some cases the improvement of one parameter was detrimental to another.