Nuclear imaging is a powerful non-invasive imaging technique that is rapidly developing in medical theranostics. Nuclear imaging requires radiolabeling isotopes for non-invasive imaging through the radioactive decay emission of the radionuclide. Nuclear imaging probes, commonly known as radiotracers, are radioisotope-labeled small molecules. Nanomaterials have shown potential as nuclear imaging probes for theranostic applications. By modifying the surface of nanomaterials, multifunctional radio-labeled nanomaterials can be obtained for in vivo biodistribution and targeting in initial animal imaging studies. Various surface modification strategies have been developed, and targeting moieties have been attached to the nanomaterials to render biocompatibility and enable specific targeting. Through integration of complementary imaging probes to a single nanoparticulate, multimodal molecular imaging can be performed as images with high sensitivity, resolution, and specificity.
1. Introduction
Molecular imaging is a non-invasive medical imaging technique capable of providing detailed images and information at molecular and cellular levels. Molecular imaging enables the visualization of cellular function through the different imaging modalities such as Positron Emission Tomography (PET) and Single-photon Emission Computed Tomography (SPECT). Imaging probes or biomarkers are used for tracking specific molecular pathways in particular targets in a living system
[1][2]. The molecular imaging techniques offer possibilities of early detection and treatments of diseases through molecular imaging. Through a variety of novel molecular imaging applications, understanding of pathological development is expected to be enhanced, facilitating drug discovery and development in tackling diseases
[3].
Bionanomaterials including organic and inorganic materials can be easily assimilated in living systems. These small-sized nanomaterials can penetrate into tiny capillaries and propagate across biological barriers, enabling detection of changes occurring at molecular levels. Combining these unique properties with being biocompatible has accelerated the application of nano-biomaterials for molecular imaging
[4]. Nanomaterials can be applied as a probe for various imaging modalities such as PET/SPECT, Computed tomography (CT), Magnetic Resonance Imaging (MRI), Ultrasound (US), optical imaging, etc. An advantage unique to nanomaterials is their large surface area-to-volume ratio, which can carry not only a large “payload” of probes at the surface but also targeting moieties or ligands, leading to a favorable biodistribution pattern of the nanomaterials in living systems. Nanomaterial probes can target areas of inflammation or tumors through passive targeting by so-called enhanced permeation and retention (EPR) effects. The imaging technique used for enhanced permeation and retention is referred to as perfusion imaging. Typically, the imaging probe is injected and monitored continuously to assess how fast the material goes into affected tissue (wash-in) and cleared from the same affected tissue (wash-out).
Nuclear imaging PET and SPECT are the modality techniques with high sensitivity. The neutron-deficient or proton-rich radioisotope undergoes positron decay, resulting in further annihilation to produce two photons that travel in opposite direction with an energy of 511 KeV. These annihilation events are collected into sinograms through tomographic techniques
[5]. The physics behind each molecular imaging technique determines the design of the nanomaterials. The nanomaterials are designed with biocompatibility for each diagnostic application. For example, the CT technique is useful for high-resolution anatomical imaging when incorporated with other tracer imaging modalities such as PET or SPECT. As the dose administered for PET and SPECT tracers are in the order of the nanomolar (nM) range, they are considered to have low toxicity and be biocompatible
[6].
Although small molecule tracers such as
18Fluorinated 2-deoxy glucose (FDG) are the most widely used clinical PET radiotracers, there is a need to develop nanomaterial-based nuclear imaging probes, as the small molecule ones exhibit fast metabolism and non-specific distribution
[7]. Nanomaterials or nanoparticles have been used as a platform to carry radiopharmaceuticals for specific targeting with other multi-functionalities. Multifunctional nanomaterials or nanoparticles have been of interest due to a number of advantages: surface modification such as coating for biocompatibility, favorable blood circulation, and easy to be manipulated for functional group attachment for enhanced targeting ability. Due to the size of nanoparticles, which are normally 100–10,000 times smaller than cells, nanoparticles can be easily tailored for cell internalization
[8]. More excitingly, the wide loading ability of nanomaterials has made it possible to simultaneously load diagnostic and therapeutic moieties into one package for theranostic applications
[9][10][11][12].
Commonly used non-invasive molecular imaging modalities include PET, SPECT, MRI, optical imaging, CT, etc. Each imaging modality has distinct advantages, while there are also inherent limitations, hindering a single imaging modality in providing all required information. Nuclear imaging techniques such as PET and SPECT are highly sensitive and provide deep penetration into tissues by using γ-ray emission; however, they are compromised by low spatial resolution
[9][11]. On the other hand, imaging modalities such as MRI and CT provide high spatial resolution but have relatively low sensitivity. Hence, the combination of different imaging modalities is favorable as a diagnostic tool for providing detailed information. For example, combining the two modalities of PET and MRI offers complementary information such as deep tissue penetration and three-dimensional (3D) anatomical information. Bimodal PET/MRI imaging is already implemented and widely used in clinical practices. Development of multimodal imaging probes can help in the identification and positioning of abnormal tissues accurately via complementary physiological and anatomical information attained from PET or SPECT and MRI, respectively.
2. Challenges of Nuclear Imaging and the Role of Nanomaterials
A key challenge faced in current nuclear imaging is the design of an imaging probe that is suitable for clinical application. An ideal imaging probe includes but is not limited to the following properties: biocompatible, easily excreted from the body, enables robust imaging signal and sensitivity, site-specific targeting to area of interest, physiochemical behavior of probe in relation to radioisotope, low toxicity, and good biodistribution
[1]. Additionally, the selection of a radioisotope for radiolabeling is also crucial in the design of the probe. There are certain criteria to consider in the development and design of an optimal probe. Sufficient half-life and optimal energy of the radioisotopes have to be complementary to the selected probes for quality imaging
[13]. For example,
68Ga and
18F for PET are radioisotopes with a short half-life utilized for imaging purposes with drugs that have fast distribution kinetics. On the other hand, longer half-life radioisotopes such as
64Cu are commonly utilized with antibodies for site-specific targeting, which requires a period of time to reach. Small molecules such as FDG are currently used as imaging probes in PET imaging. However, small molecules tend to exhibit non-specific distribution and poor uptake in certain tumors, which could lead to deviation from diagnostic results
[2][7][14].
Nanomaterials can potentially overcome these limitations due to their advantageous size and physiochemical properties
[4]. The nanosized feature allows localization of probes at disease sites and reduction in renal excretion and metabolism in the liver due to the unique properties of EPR, enabling prolonged circulation in the body
[15]. The pharmacokinetic behavior and biodistribution of the nanomaterial probes allow their theranostic applications with regard to various preclinical and clinical objectives.
The limitations of crossing biological barriers such as the blood–brain barrier (BBB) and small capillaries can also be overcome using nanomaterials. These nanosized vessels are small enough to propagate across the barriers, delivering drugs to targeted sites
[4].
3. Modifications of Nanomaterials for Nuclear Imaging
To improve their biocompatibility, targeting specificity, and easily controllable properties, nanomaterial-based nuclear imaging probes require necessary surface modifications such as coating and targeting moiety attachments, which include conjugation with peptides, antibodies, and many other targeting molecules (
Scheme 1). The modifications promote various advantageous properties such as site-specific targeting, higher affinity to biomolecules, enhanced uptake into cells, improved biocompatibility, and longer circulation time that are essential for theranostic applications.
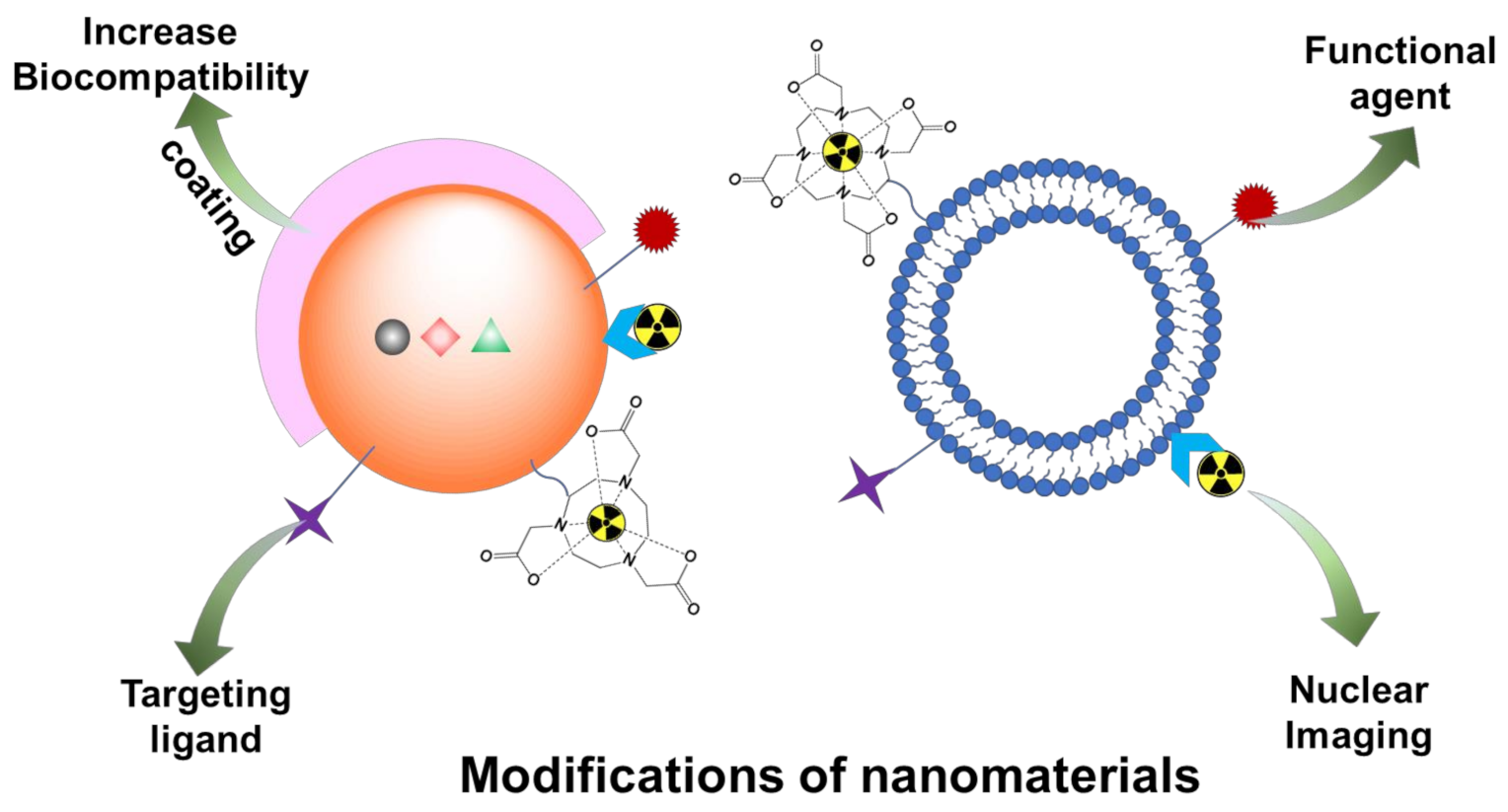
Scheme 1. Various modifications of nanomaterials as nuclear imaging probes for their multi-functional theranostic applications.
3.1. Coating
The nanomaterial probe administered intravenously into the living system is assimilated either by the hepatobiliary system or by the renal system. To improve the biocompatibility, the nanoparticles are coated with hydrophilic polymers that include linear chain polymers of polyethylene glycol (PEG) and branched copolymers
[16][17].
Apart from PEG, several other coating polymers including polyvinyl pyrrollidone (PVP)
[18], polyvinyl alcohol (PVA)
[19], polylactide, polycaprolactone, polaxomers of polyethylene oxide (PEO), and polypropylene oxide (PPO) have been developed.
3.2. Active Targeting Moieties for Disease-Specific Receptors
Nanomaterial itself in which the radiolabeled isotopes are packed and delivered to the living system is essential for non-specific uptake and ideal distribution behavior for molecular imaging. The nano-sized particles offer a greater surface area per volume of the particle, giving accessibility for functionalization of the surface with ligands including biological peptides, antibodies, vitamins, and other biological molecules. These nanoparticles can selectively bind to cell surface receptors and cause specific “signal” changes that can be detected. The “signals” are due to the behavior of the nanoparticle. Targeting moieties have been designed to bind to receptors that are over-expressed in pathological cells but much less expressed in normal cells
[20][21].
4. Nanomaterials for Theranostic Nuclear Imaging Probes
Radiolabeled peptide or antibody can be incorporated onto nanoparticles or nanomaterials as PET (SPECT) imaging probes for cancer diagnostics as these nanoparticles demonstrated high affinity and selectivity for receptors that are over-expressed by various human cancers such as breast, lung, and prostate tumors
[7][22][23].
Liposomes, especially PEGylated long-circulating liposomes, have been widely used as nanocarriers for drug delivery. Ranging from 50–1000 nm, these sphere vessels composed of lipid bilayers surrounding the aqueous core have shown great potential as theranostic nuclear imaging probes
[24].
PET radioisotopes can be incorporated into quantum dots (QDs) to produce optical/PET bimodal probes. These nanomaterials with quantum confinement present unique properties for in vivo imaging studies such as biodistribution of QDs to image cancer
[25][26].
5. Radiolabeled Exosomes for Nuclear Imaging
Exosomes were first discovered in the 1980s when it was hypothesized that the debris of these nanosized vesicles was secreted into the extracellular space from cells during blood reticulocyte maturation to remove unwanted transferrin receptors
[27][28][29][30][31][32]. Until 1996, these exosomes were considered debris, but since then, studies have reported the role of exosomes in immune responses such as antigen presentation in immune-modulating activity of B cell-derived vesicles and their potential in immunotherapy
[30][33][34][35].
Since exosomes encapsulate many bioactive materials important for paracrine factors, tumor growth, and suppression, these vesicles can serve as biomarkers for diagnosis and prognosis for diseases
[31][35][36][37]. Studies have shown that exosome concentrations were found to be higher in the blood of patients with cancer
[31][34][35]. Additionally, radiolabeled exosomes can also be used for tracking of pharmacokinetics via different routes of administration, which is important in biodistribution studies in determining the design of the drug
[38][37][39] for clinical translation.
6. Conclusions
With the development of nuclear imaging probes using nanomaterials, pathological processes at molecular levels can be revealed that could potentially help in early detection and interventions of various diseases. Enhancement of nanomaterial targeting capabilities as imaging probes has been explored through various approaches such as surface modifications and targeting moieties attachment. The applications of nanomaterials have been extensively exploited for biocompatibility, in vitro stability, and in vivo biodistribution and animal imaging. Despite promising results from in vivo studies, there are still challenges that need to be overcome before nanomaterials can be applied as diagnostic and/or therapeutic nuclear medicine and imaging tools in clinical applications. For example, the reproducibility of various nanomaterials needs to be systematically determined, and the synthetic methods need to be standardized to ensure their biocompatibility so that theoretical modelling of nanomaterial integration can be guided for cell tracking and cell internalization.