Hydrogen energy, as a clean and renewable energy, has attracted much attention in recent years. Water electrolysis via the hydrogen evolution reaction at the cathode coupled with the oxygen evolution reaction at the anode is a promising method to produce hydrogen. Given the shortage of freshwater resources on the planet, the direct use of seawater as an electrolyte for hydrogen production has become a hot research topic. Direct use of seawater as the electrolyte for water electrolysis can reduce the cost of hydrogen production due to the great abundance and wide availability. Various high-efficiency electrocatalysts have made great progress in seawater splitting and have shown great potential.
1. Introduction
Due to the ever-increasing energy demand and the exponential growth of energy consumption, people are facing a great challenge caused by the shortage of fossil energy storage together with the related pollution. The energy conversion system, to generate green energy as an alternative to fossil fuels, becomes an essential part to build a roadmap towards sustainable energy future
[1][2]. Among the green and sustainable energy, hydrogen is a clean energy resource with great abundance and significant energy density, since it can be produced from water without release of carbon dioxide or other toxic products in the process of conversion to other forms of energy. Fossil fuels (such as natural gas, coal, or biomass gasification) are still the main source for producing hydrogen in the current industrial production, occupying 96% of hydrogen production. Only 4% is produced through electrolytic water
[3][4][5]. Theoretically, only a potential of 1.23 V is required for water splitting. However, a high voltage, typically larger than 1.8 V, is needed to trigger the water splitting reaction. Water electrolysis consists of a two-electron transfer cathodic hydrogen evolution reaction (HER) and a four-electron coupled anodic oxygen evolution reaction (OER). The large activation barriers in HER and OER pathways result in large overpotentials, thus leading to slow kinetics of water electrolysis
[6][7][8]. Therefore, people must use electrocatalysts with high activity to reduce the activation energy barrier of HER and OER, lower the overpotential and ultimately achieve a more efficient energy conversion.
At this stage, research of electrolysis for hydrogen production using freshwater has yielded good results; however, research of seawater electrolysis for hydrogen production is still at the early stage
[9][10]. The freshwater resource is scarce, most of which remains frozen or in the form of chemosynthetic water. On the contrary, seawater, accounting for 96.5% of the earth’s resources, is world-widely abundant. It is promising to utilize seawater as electrolyte for water electrolysis to produce hydrogen. However, there are up to 3.5 wt% salts in seawater in presence of different metal ions, which may participate in various competing electrochemical reactions to HER at the cathode, limiting the efficiency of water electrolysis
[9][10][11][12]. Moreover, the presence of bacteria and microorganisms in natural seawater can lead to the formation of insoluble precipitates on the active site of the catalyst surface, which may affect the HER performance (
Figure 1)
[13][14]. On the other hand, high concentration of chloride ions (ca. 0.5 M) may block the active center of catalysts
[15]. Furthermore, the chlorine evolution reaction (CER) may also occur as a competitive reaction to OER at the anode
[16]. Under acidic conditions at pH = 0, the theoretical overpotential of four-electron transfer OER (1.23 V vs. SHE) is 130 mV lower than that of two-electron transfer CER, and thus, CER has faster kinetics
[17]. Given that the overpotential of CER does not vary with pH as OER does, the inhibition of CER can be achieved by increasing the alkalinity of the electrolyte. Nevertheless, another reaction occurs in an alkaline environment, namely, the hypochlorite production reaction
[18]. Therefore, in order to achieve high-efficiency hydrogen production and realize industrial seawater electrolysis, it is necessary to have a systematic summary and in-depth understanding of the reaction mechanism as well as the related parameters affecting the performance.
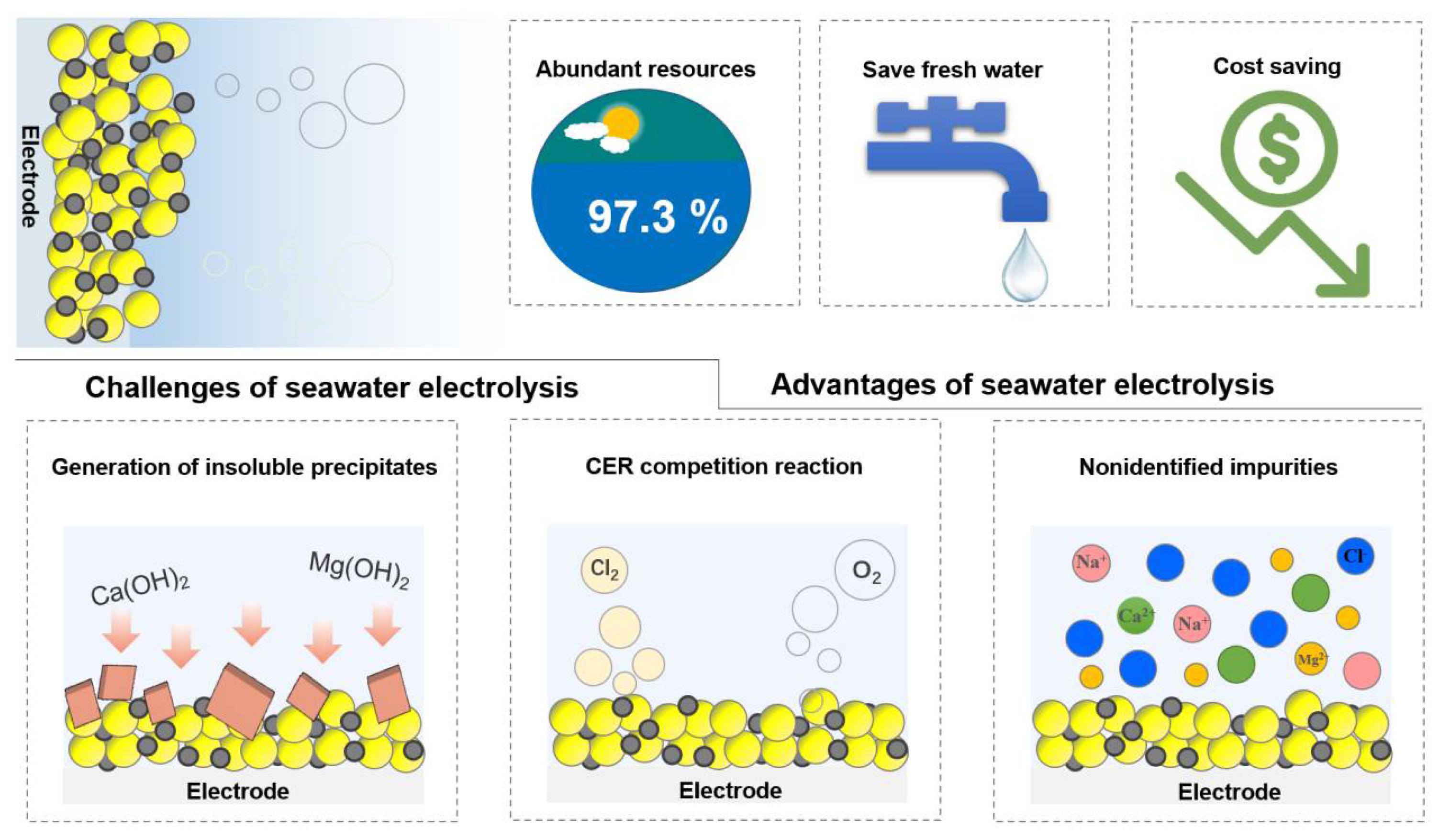
Figure 1. Advantages and challenges of seawater electrolysis.
Up to now, various catalysts have been studied into the seawater electrolysis
[9][10][19][20][21][22][23][24][25][26][27][28][29][30][31][32]. Successful examples, such as metal (hydrogen) oxides, nitrides, phosphides, borides, and hybrid catalysts have been applied as catalysts for OER in seawater. In addition to what have been studied for OER, noble metal alloy, carbon-supported noble metals, MXene-based complexes as well as the hybrid materials with different composites or structures have been utilized as catalysts for HER in seawater. All these catalysts have their own merits and demerits in the seawater electrolysis.
2. Electrocatalysts for Driving Seawater Electrolysis
2.1. Electrocatalysts for OER
As discussed above, the competitive oxidation of chloride ions in seawater not only reduces the efficiency of the OER but also produces chlorine-containing species damaging the electrolyzer and causing environmental issues
[33][34][35]. Consequently, excellent catalysts are needed to enable the selective OER. Up to now, a large number of materials, such transition metal oxides and hydroxides, metal phosphides, metal nitrides, metal borides, and hybrid electrocatalysts, have been explored as selective OER catalysts in seawater electrolysis (
Table 1).
Table 1. Summary of OER performance of reported electrocatalysts.
2.2. Electrocatalysts for HER
Previous studies have shown few competitive reactions to the HER in seawater electrolytes, unlike those in the anode compartment. However, the main problem of cathodic HER is the presence of impurities in seawater, which leads to blockage and corrosion of the active sites, resulting in low efficiency and poor stability
[45]. At this stage, mostly reported electrocatalysts for HER in seawater electrolysis include noble metal alloys, carbon-supported noble metals, MXene based complexes, metal phosphides, metal oxides, metal hydroxides, metal nitrides, hybrid electrocatalysts, and so on (
Table 2).
Table 2. Summary of HER performance of reported electrocatalysts.
3. Conclusions and Outlook
Seawater is an inexhaustible resource on the planet; thus, the hydrogen production through the electrolysis of seawater can be effective in alleviating the energy crisis to some extent. Despite many efforts devoted into the field of seawater electrolysis in recent years, there is large space for the improvement of seawater electrolysis for high-performance production of hydrogen. Obviously, seawater electrolysis is more complex than freshwater electrolysis due to the presence of multiple cations and anions. Herein concluded the recent progress and summarize in detail the current status of research on HER and OER catalysts for seawater electrolysis, from fundamental mechanisms to the performance of catalysts.
Extensive research has been carried out on various materials, such as metal oxides (hydroxides), metal nitrides, metal phosphides, metal borides, noble metal alloy catalysts, and so on, for seawater electrolysis. Still, the catalytic activity and stability of most of the reported catalysts are not satisfactory to meet the requirement for practical application. As can be found from Table 1 and Table 2, the overpotential of these catalysts is not low enough. Furthermore, most of the electrolytes for current research are artificial saline water rather than real seawater, which increases the complexity of seawater electrolysis for hydrogen production. Therefore, in order to achieve high-performance seawater electrolysis, it is believed that more efforts are needed in the following directions:
- (1) Combining experimental and theoretical analyses to further confirm the reaction pathways and active sites of catalysts for HER and OER in seawater electrolysis: in addition to monometallic compounds, various polymetallic compounds and heterostructured catalysts have been extensively investigated as catalysts in seawater electrolysis. It is a trend to design catalysts composed of more than a single metal component by taking advantage of the synergistic effect of multimetal components. As catalyst components become more complex, it is more difficult to identify the electrocatalytic reaction pathways as well as the active sites. Therefore, systematic research based on theoretical analyses is necessary, which can provide guidance for designing materials with desirable structures and properties.
- (2) Employing in situ characterization methods to unravel the true active sites of catalysts: many current electrocatalysts, such as metal oxides, phosphides, and nitrides, undergo surface oxidation or reconstitution during seawater electrolysis, which means that the true active sites of the catalyst may be altered during the reaction. As each step in the catalytic reaction process changes rapidly, scientists need in situ characterization techniques to track changes in the intermediates during the catalytic reaction process. It will provide clear principle and guidance to design high-efficiency catalysts if more in situ techniques such as in situ XAS, Raman, Fourier transform infrared spectroscopy, and other novel techniques are involved for the mechanistic studies.
- (3) Exploring and developing electrocatalysts with high activity and stability in seawater: Not only multiple cations but also chloride anions in seawater interfere with the water splitting reactions. It is highly desirable to synthesize catalysts with higher selectivity to HER and OER than other competitive reactions. To obtain highly efficient electrocatalysts for seawater electrolysis, modulating the electronic structure of active sites is of great significance and plays a major role in the improvement of catalytic performance. To optimize the electronic structure of catalysts, alloying, vacancy engineering, heteroelement doping, and interface engineering are common methods. Integration of different active materials into a hybrid catalyst is also a good solution for developing high-performance catalysts.
- (4) Designing advanced reactors specific for seawater electrolysis: The current research of seawater electrolysis for hydrogen production is mostly focused on the catalysts. To realize the electrocatalytic production of hydrogen, herein need to consider the entire reactor rather than the catalysts only. It is necessary to reasonably design reactors which are adaptable to specific seawater electrolysis. For example, the design of asymmetric reactors is considered to be more promising [31][59], which consists of alkaline water in the anode chamber and seawater in the cathode chamber. Such design not only facilitates the diffusion of Cl− to the anode but also protects the anode catalyst, which is of significance in seawater electrolysis.