Plant and animal cells produce various oxidative compounds, such as H
2O
2, O
2−, and OH
−, which can damage lipids, proteins, and DNA and can induce several health complications including cancer, aging, and neurological disorders in humans
[55]. However, another group of compounds, known as antioxidants, has the potential to counter these effects
[56][57]. Lemongrass possesses antioxidants that render protective measures against reactive species
[58][59]. Lemongrass extracts have been reported to reduce reactive species concentration, lipid peroxidation, and decolourisation of 2,2-diphenyl-1-picrylhydrazyl
[60][61]. Lemongrass extract can also buttress the endogenous antioxidant defence system in alveolar macrophages cells through augmenting superoxide dismutase activity and glutathione formation
[61][62]. A vast array of plant extracts has been studied for their beneficial antioxidant properties. In particular, plant antioxidant potential may protect cells and organs from radical oxygen species. The natural extracts are usually compared to controls, such as butylated hydroxy anisol (BHA) or buthylhydrotoluene (BHT), for their antioxidant profile. Radical scavenging activity is evaluated by their activity towards a stable free radical, 2,2-azino-bis-3-ethylbenzothiazoline-6-sulphonic acid (ABTS). To date, there are no published reports on the antioxidant power of LEO. These data could be useful in comparing different cultivars or different methods of cultivation. Plant extracts have been studied, on cultured cells, for their antioxidant properties, their enzymes, and their redox potentials. In particular, regulation of redox status in cells may affect the levels of methyl group donor S-adenosylmethionine (SAM). SAM is a cofactor for histone methyltransferases and DNA methyltransferases. The consumption of glutathione, as occurs during oxidative stress, with an increase of its oxidized form, glutathione disulfide, may inhibit S-adenosylmethionine (SAM) synthetase, with a reduction of SAM synthesis
[63] influencing the epigenetic modifications of proteins and DNA. Citral was shown to increase intracellular oxygen radicals, while inhibition of glutathione synthesis increased citral’s anticancer effect
[63]. Citral was shown to modulate oxidative stress preferentially in cancer cells and to induce the endoplasmic reticulum stress exerting thus an antiproliferative action
[64]. Lemongrass has a competitive advantage over other synthetic antioxidants, such as butylated hydroxytoluene, since they can induce haemorrhage: in this view, lemongrass oil is regarded as ‘safe’ for human consumption
[64]. This opens a new vista for lemongrass oil in the food preservation and safety industries, including the meat, and dairy industries
[41]. In the food industry, the oxidation of lipids is an important determinant of meat and dairy products. However, their highly rich nutritional profiles are prone to lipid peroxidation and quality deterioration. In this regard, coating such products with LEO minimizes lipid peroxidation and increases their shelf life and quality
[65]. Furthermore, the antioxidant nature of citral is exploited in animal skin cancer models
[66][67]. Soares et al.
[68] reported that LEO, which was characterised in its major components, showed high antioxidant activity compared to the methanolic and aqueous extracts of lemongrass. In detail, they showed higher antioxidant capacity of LEO compared to the aqueous extracts of leaves: Using the 2,2-diphenyl-1-picrylhydrazyl free radical as a control, they obtained similar antioxidant power, at levels of 41 μg/mL. The antioxidant activity of LEO can further be enhanced through mixing it with other potent antioxidative agents. On this note, a mixture of LEO with
Ocimum gratissimum, and
Thymus vulgaris oil had enhanced effects against
Bipolaris oryzae and
Alternaria alternata [68][69]. A review on the antioxidant, antimicrobial and antifungal properties of LEO and recent updates on the possible applicative uses has been recently published
[70].
3. Anticancer Activity
According to the World Health Organization (WHO), cancer caused an approximated 10 million deaths, or one in six deaths, in 2020. This situation is not going to be relieved, as there is estimated to be an increase of 45% in cancer mortality rate between 2008–2030. Among cancers, the most common types are breast cancer, lung cancer, colorectal cancer, prostate cancer, skin, and stomach cancer. The ongoing conventional chemotherapies, radiotherapy treatment, and surgeries have shown a large number of involuntary side effects due to insufficient knowledge of treatment specificity, and are not recommended for long-term usage
[71][72].
Medicinal plants emerge as potential candidates in the cancer world and raise hopes for the scientific community. Scientists are constantly looking for natural sources to uncover the potential plant-based therapeutic agents having immense anticancer properties
[73]. In this sense, the essential oil of lemongrass counts among such plants for its cytotoxicity on human cancer cells. Its active ingredients, including geraniol, geranyl acetate, α-bisabolol, and iso-intermedeol have individually been found to impart cytotoxic effects on cancer cells
[74]. Lemongrass EO has exhibited inhibition of human mouth epidermal carcinoma (KB) and murine leukemia cell lines (P388)
[75].
Among LEO terpenes, citral, the major component of lemongrass oil, plays a potential role as antiproliferative against several types of cancer cells, such as the two human prostate cancer cell lines, LNCaP and PC-3
[74], HL60, U937 ovarian cancer cells
[75][76][77], cervical cancer cell lines
[78], and the breast cancer cell line, MCF-7
[79]. Interestingly, citral does not exert cytotoxicity to normal epithelial cells but exhibits toxic effects against human breast cancer cell lines, confirming its cancer-specific efficacy
[80].
Although a large number of studies evinced the anticancer activity of lemongrass, scarce data are available on its mode of action. Studies based on different cancer cell types substantiated citral efficacy via activated procaspase-3, induction of apoptosis, and cell cycle arrest in the G2/M phase
[81][82]. Citral consists of a double bond in conjugation with an aldehyde (α, β-unsaturated) group in its core structure, which serves as a potent caspase 3 activator, responsible for pro-apoptotic activity
[81]. Moreover, citral-induced apoptotic activity was associated with DNA fragmentation and induced caspase 3 activity against hematopoietic cancer cell lines and ovarian cancer cell lines.
The Src-tyrosine kinase is expressed in small cell lung cancer and can phosphorylate transcription factor Stat3(Y705)
[81], which sequentially enhances the expression of downstream genes engaged in the antiapoptotic activity, i.e., Bcl-xL and Mcl-1
[83]. An experimental study showed the inhibitory effects of LEO and citral on phosphorylation of Src(Y416) blocking its activation, resulting in reduced phosphorylation of Stat3 (Y705). Non-phosphorylated Stat3 disrupts cell growth and the signal pathways that upregulate the expression of Bcl-xL and Mcl-1
[84]. Citral-dependent apoptosis induction has also been observed against prostate cancer cell lines. Citral induced gene activation initiates AMPK (an enzyme necessary in the fatty acid metabolism) phosphorylation resulting in the activation of BAX and the downregulation of Bcl-2, which initiates an apoptosis cascade in prostate cancer cell lines
[85]. Citral-mediated breast tumour growth inhibition via the inhibition of ALDH1A3 was reported
[86]. The up-regulation of retinoic acid (RA) signalling by ALDH1A3 can cause breast cancer growth, and citral inhibited the expression of RA-inducible genes mediated by ALDH1A3
[86]. Microtubule affinity regulating kinase 4 (MARK4), an AMP-activated protein kinase
[87], is reported to mediate apoptosis, inflammation, and distinct regulatory pathways
[88]. Alterations in MARK4 expression hamper the cell cycle and eventually cause cancer. Citral potentially binds to MARK4 and inhibits its kinase activity, and is being considered an effective strategy to prevent the growth of cancer cells and other MARK4 associated diseases
[89]. Citral has been reported inducing the phosphorylation of p53 protein and the expression of Bax, while reducing the expression of the antiapoptotic factors Bc-2 and Bcl-xL in human colorectal cancer lines, i.e., HT116 and HT29
[90]. Citral interferes with the ERK1/2 pathway and reduces the translocation of ERK1/2 protein to the nucleus. There is a certain possibility of the involvement of ERK1/2 in melanoma carcinogenesis and the progression in presence of mutated
N-Ras and
B-Raf. Therefore, citral could negatively affect cancer growth by inhibiting the final step of the MAPK cascade
[91].
Geraniol, the second major constituent of LEO, has garnered heed for its potentiality in cancer treatment. It has been reported that geraniol induces the production of ROS and inhibits the phosphorylation of tyrosine kinases, which, in turn, induce apoptosis of cancer cells
[92]. Several studies have been conducted to gain insights into its anticancer activity
[93][94][95]. Ornithine decarboxylase (ODC) plays a prime role in the synthesis of polyamines, providing stabilization to DNA structure
[96]. A decrease in ODC activity after geraniol treatment has been observed in the intestinal adenocarcinoma Caco-2 cell line, which, in turn, caused DNA synthesis inhibition and cell cycle arrest in the S phase
[96]. Polyamine metabolism is a potential target in the development of cancer-preventive drugs, therefore, geraniol mediated decline in ODC activity might have a useful clinical role
[97]. Geraniol-induced inhibition of the proliferation of A453 and A549 human lung cancer cell lines has been reported. Geraniol alters the tubulin polymerization and disrupts the active property of both the studied cell lines, resulting in cell apoptosis. Geraniol arrested the G0/G1 phase in A431 cells, with no effects on sub-diploid cells, and the G2/M phase of A549 with the increased population of sub-diploid cells, in a dose-dependent manner. The inhibitory effects of geraniol might be interrelated with the observed alteration in the ODC activity
[98]. Geraniol caused inhibition of cell cycle progression, exerting altered expression of cyclins D1, A, B1, CDK2, and the cyclin kinase inhibitor proteins p21 and p27
[99]. Geraniol has been reported to induce the expression of pro-apoptotic proteins Bcl-2, Bax, Bak, and caspase3/8/9 in several human cancer cell lines
[95][100]. Moreover, the considerable increase in these proteins indicates that geraniol induces apoptosis through the mitochondrial intrinsic pathway
[101]. The antiangiogenic activity of geraniol has been confirmed by both in-vivo and in-vitro studies. Geraniol suppresses the endothelioma cell line and reduces Ki67-positive cells and CD3-microvessela by suppressing the expression of VEGFR-2 in Balb/c mice
[102]. This activity might play a role in reducing tumour growth, as the tumour needs a new blood vessel to grow. Geraniol arrested the proliferation of two pancreatic cancer cell lines, MIA PaCa-2 and BxPC, in hamsters when injected with PC-1 pancreatic ductal adenocarcinoma cells. In both the cell lines, it arrested the G1 phase of the cell cycle along with increasing the expression of the cyclin kinase inhibitor proteins p21
cip1 and p27
kip1 while suppressing those of cyclin A, B1, and CDK2
[103][104].
Figure 1 indicates a series of distinctive signalling pathways activated in cancer cells via different components of LEO.
Figure 1. Distinctive signalling pathways activated in cancer cells via different components of LEO. Every component acts differently against cancer cells and involves diverse signalling pathways. All the involved pathways lead to inhibition of cell migration, cell cycle, and DNA synthesis. All these events eventually cause cell death (apoptosis). MARK4, Microtubule affinity-regulating kinase 4; AMPK, 5′ adenosine monophosphate-activated protein kinase; BAX, BCl2- associated X protein; BCL2, B-cell lymphoma 2; BID, BH3-only activator protein; tBid, truncated Bid, ROS, reactive oxygen species; ODC, ornithine decarboxylase; VEGF, vascular endothelial growth factor; VEGFR, vascular endothelial growth factor receptor; AKT, Ak strain transforming; ERK, extracellular regulated kinase; TNF-α, tumour necrosis factor; RIP1, receptor-interacting serine-threonine protein kinase 1; RIP3, receptor-interacting serine-threonine protein kinase 3; PARP, poly ADP ribose polymerase; DNA, deoxyribonucleic acid.
D-limonene, another constituent of LEO, was also reported to possess antineoplastic activity. D-limonene enhances the activity of carcinogen metabolizing enzymes, such as cytochrome P450, responsible for the conversion of carcinogens into less harmful forms and blocks their interaction with DNA
[105]. Treatment of D-limonene on LS174T human colon cancer cells inhibited the P13K/Akt pathway and induced cell apoptosis. An increase in PARP cleavage and activation of caspase-3 indicates the involvement of the mitochondrial apoptotic pathway
[106]. Limonene-mediated induction of apoptosis via increased expression of Bax and caspase-3 and decreased Bcl-2 expression has been reported in T24 bladder cancer cells. Moreover, limonene arrested the cell cycle in the G2/M phase and wound healing and transwell assay using Matrigel has confirmed the limonene mediated suppression of cancer cell migration and invasion
[107]. Another component of the EO of lemongrass, citronellol, is also found to exert cytotoxic effects on several cancer cell lines
[108][109][110]. Citronellol showed its anticancer activity via increased reactive oxygen species production, alterations in mitochondrial permeability, DNA fragmentation, changes in cytochrome c activities, and activation of caspase, against the MCF-7 human mammary tumour cell line
[111]. The cytotoxicity of α-bisabolol has been reported against human and rat malignant glioma cancer cell lines. In α-bisabolol-treated cell lines, the rapid loss of inner transmembrane potential and an increase in cytochrome-c translocation indicate that α-bisabolol can trigger apoptosis through mitochondrial intrinsic pathway
[112]. Another experimental study has confirmed the cytotoxic effect of α-bisabolol against several cancer cell lines. α-bisabolol arrested cell cycle and initiated cancer cell death via a BID (BH3-only activator protein)-dependent mechanism
[113]. It induces the permeability of the outer mitochondrial membrane and plays a crucial role during apoptosis
[114]. α-bisabolol-induced damage to lysosomal and mitochondrial membranes via BID resulted in autophagy and regulated cell death, enlightening its modes of action
[113][114][115][116].
All of the mentioned components of LEO have presented their chemo-preventative effects via arrest of different phases of the cell cycle, suppression of cyclins and cyclin-dependent kinases, DNA fragmentation, and antiangiogenic activity, against different cancer cell lines. Several distinct signalling pathways and mechanisms of action have been reported in experimental studies exhibiting the anticancer activities of these mentioned component.
Epigenetic modifications play a key role in cancer proliferation. Several chromatin remodelling complex components are found mutated, silenced, or overexpressed in cancers. Epigenetic mechanisms may be taken into account to explain the regulation of various protein-coding and protein non-coding genes. One type of regulation of gene transcription is based on the level of DNA methylation on promoters, controlled by DNA methyltransferases. A second mechanism is dependent on chromatin accessibility mediated by remodelling complexes, recruitment of Polycomb repressing complexes, and histone-modifying enzymes
[63]. An involvement of non-coding RNAs (nc-RNAs) is at the basis of these mechanisms; therefore, the expression of long and small non-coding RNAs determines whether an antioncogenic pathway is repressed or downregulated, or oncogenes are set free to induce cell transformation. Therefore, chromatin accessibility (opening or compaction), access to transcription machinery, and promoter methylation are the principal mechanisms that are targeted by plant extracts, essential oils, and individual bioactives. Various classes of bioactives were shown to exert anticancer effects through the downregulation of microRNAs, most often oncomiRs, and the release of mRNAs coding for antiproliferative proteins. The review by Sabo
[117] and that by Cherng
[118] showed the relationship between natural compounds and nc-RNAs in cancer cells, and the potential use of their bioactives in cancer therapies. Xu and colleagues described, for the first time, the involvement of pinene, one component of LEO, in the upregulation of p27/CDKN1B, a cell cycle-blocking protein, through the downregulation of miR-221
[118]. The most well-known group of nc-RNAs are microRNAs
[119]. When they are abundant, they silence mRNA transcription by sequestering them and destining them to degradation. Long non-coding RNAs, such as competing endogenous RNAs (ceRNAs), may sponge a group of miRNAs and the relative abundance thereof determines whether the miRNA can exert its effects or is bound to the sponge
[119]. This has been clearly reviewed for stilbenes
[120] and other plant bioactives
[120][121].
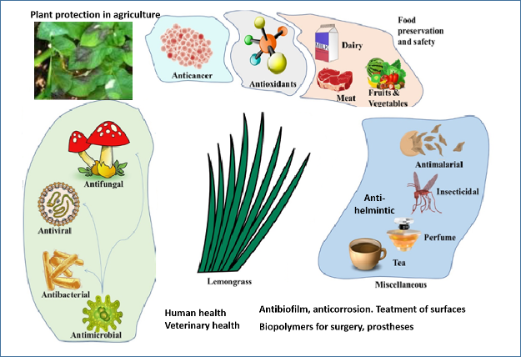
In conclusion, the bioactive LEO phytocomponents are useful for a myriad of medicinal properties including anti-microbial, anticancer, antioxidant, insecticidal, and antimalarial activities (Figure 2). Although LEO and its constituents have shown anticancer activity in vitro, and, in some cases, in animal studies, only a few researchers to date have tested the delivery of its bioactive components combined with nanoparticles or delivery systems. a variety of applications, from food safety and food preservation, in terms of antioxidant potential as well as for antifungal properties, to applications in agriculture and veterinary medicine, and as coatings on biopolymers for surgery (maxillofacial silicone specimens in dentistry, other medical implants) have been recently proposed. The ability of LEO terpenes to stop bacteria and fungi from growing in biofilms has also a wide array of applicative uses in medicine and surgical devices, and in industrial solutions to biocorrosion, biofouling, biodegradation, water microbiology, and the control of bacterial quorum sensing signals [103]. The ability to modify the materials used in medical devices allows the application of LEO components to make such surfaces resistant to biofilm formation.